Upregulated Connexin 43 Induced by Loss-of-Functional S284L-Mutant α4 Subunit of Nicotinic ACh Receptor Contributes to Pathomechanisms of Autosomal Dominant Sleep-Related Hypermotor Epilepsy - PubMed
- ️Wed Jan 01 2020
Upregulated Connexin 43 Induced by Loss-of-Functional S284L-Mutant α4 Subunit of Nicotinic ACh Receptor Contributes to Pathomechanisms of Autosomal Dominant Sleep-Related Hypermotor Epilepsy
Kouji Fukuyama et al. Pharmaceuticals (Basel). 2020.
Abstract
To study the pathomechanism and pathophysiology of autosomal dominant sleep-related hypermotor epilepsy (ADSHE), this study determined functional abnormalities of glutamatergic transmission in the thalamocortical motor pathway, from the reticular thalamic nucleus (RTN), motor thalamic nuclei (MoTN) tosecondary motor cortex (M2C) associated with the S286L-mutant α4β2-nicotinic acetylcholine receptor (nAChR) and the connexin43 (Cx43) hemichannel of transgenic rats bearing the rat S286L-mutant Chrna4 gene (S286L-TG), which corresponds to the human S284L-mutant CHRNA4 gene using multiprobe microdialysis, primary cultured astrocytes and a Simple Western system. Expression of Cx43 in the M2C plasma membrane fraction of S286L-TG was upregulated compared with wild-type rats. Subchronic nicotine administration decreased Cx43 expression of wild-type, but did not affect that of S286L-TG; however, zonisamide (ZNS) decreased Cx43 in both wild-type and S286L-TG. Primary cultured astrocytes of wild-type were not affected by subchronic administration of nicotine but was decreased by ZNS. Upregulated Cx43 enhanced glutamatergic transmission during both resting and hyperexcitable stages in S286L-TG. Furthermore, activation of glutamatergic transmission associated with upregulated Cx43 reinforced the prolonged Cx43 hemichannel activation. Subchronic administration of therapeutic-relevant doses of ZNS compensated the upregulation of Cx43 and prolonged reinforced activation of Cx43 hemichannel induced by physiological hyperexcitability during the non-rapid eye movement phase of sleep. The present results support the primary pathomechanisms and secondary pathophysiology of ADSHE seizures of patients with S284L-mutation.
Keywords: connexin; hemichannel; idiopathic epilepsy; microdialysis; zonisamide.
Conflict of interest statement
The authors state no conflict of interest.
Figures
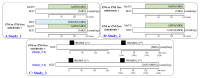
Schematic experimental designs of microdialysis experiments. Panels A, B, C are represented the experimental designs of Study_1, Study_2 and Study_3, respectively. MRS: modified Ringer’s solution, HKMRS: 100 mM potassium containing MRS, RJR2403 (100 μM: selective α4β2-nAChR agonist): (E)-N-Methyl-4-(3-pyridinyl)-3-buten-1-amine oxalate, AMPA (100 μM: AMPA/glutamate receptor agonist): amino-3-(3-hydroxy-5-methyl-isoxazol-4-yl)propanoic acid, GAP19 (100 μM: selective connexin43 (Cx43) hemichannel blocker), MoTN: motor thalamic nuclei, RTN: reticular thalamic nucleus, M2C: secondary motor cortex.
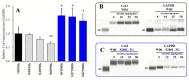
Dose-dependent effects of subcutaneous administration of subchronic doses of nicotine (0, 10, 25 and 50 mg/kg/day) for 7 days on Cx43 expression in the M2C plasma membrane fraction of wild-type and S286L-TG (A) and pseudo-gel images using Simple Western using anti-GAPDH and anti-Cx43 antibody for blotting of plasma membrane fractions of wild-type (B) and S286L-TG rats (C). In panel 2A, ordinate: mean ± SD (n = 6) of relative protein level of Cx43. *P < 0.05, **P < 0.01 relative to nicotine free wild-type by one-way analysis of variance (ANOVA) with Tukey’s post hoc test.
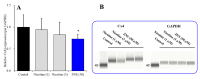
Concentration-dependent effects of subchronic administration of nicotine (0, 1 and 3 μM) and lowest therapeutic-relevant concentration of zonisamide (ZNS) (50 μM) for 7 days on Cx43 expression in the plasma membrane fraction of primary cultured astrocytes from wild-type rat (A) and pseudo-gel images using Simple Western results using anti-GAPDH and anti-Cx43 antibody for blotting of plasma membrane fractions of wild-type (B). In panel 3A, ordinate: mean ± SD (n = 6) of relative protein level of Cx43. *: P < 0.05 relative to control by student T-test. Concentration-dependent effects of nicotine on Cx43 expression was not detected by one-way ANOVA.
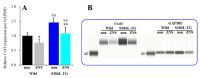
Effects of systemic subchronic administration of ZNS (40 mg/kg/day for 7 days) on Cx43 expression in the M2C plasma membrane fraction of wild-type and S286L-TG (A) and pseudo-gel images using Simple Western results using anti-GAPDH and anti-Cx43 antibody for blotting of plasma membrane fractions (B). In panel 4A, ordinate: mean ± SD (n = 6) of relative protein level of Cx43. *P < 0.05, **P < 0.01 vs. ZNS-free (non) and @@P < 0.01 vs. wild-type by two-way analysis of variance (ANOVA) with Tukey’s post hoc test.
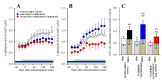
Effects of local administration of RJR2403 (α4β2-nAChR agonist) into the reticular thalamic nucleus (RTN) and subchronic administration of therapeutic-relevant dose of ZNS (40 mg/kg/day for 7 days) on the L-glutamate release in the secondary motor cortex (M2C) induced by local administration of 100 μM amino-3-(3-hydroxy-5-methyl-isoxazol-4-yl)propanoic acid (AMPA) into the motor thalamic nuclei (MoTN) of wild-type (A) and S286L-TG (B). Rats were subchronically administered a therapeutic-relevant dose of ZNS (chronic ZNS) or ZNS-free (control or AMPA+RJR) using a subcutaneous osmotic pump. In ZNS non-treated rats, perfusion medium in the RTN was commenced with modified Ringer’s solution (MRS) with (AMPA+RJR: blue circles) or without (control: opened circles) 100 μM RJR2403 (blue bars). After the stabilization of L-glutamate levels in the M2C, the perfusion medium in the MoTN was switched from MRS to MRS containing 100 μM AMPA for 180 min (gray bars). In ZNS treated rats (chronic ZNS: red circles), perfusion medium in the RTN was commenced with MRS containing 100 μM RJR2403. After the stabilization of L-glutamate levels in the M2C, the perfusion medium in the MoTN was switched from MRS to MRS containing 100 μM AMPA for 180 min (gray bars). Ordinates indicate mean extracellular L-glutamate level (μM) (N = 6), and abscissas indicate time after AMPA-evoked stimulation (min). Blue bars indicate the perfusion with 100 μM RJR2403 into the RTN. Gray bars indicate the perfusion with 100 μM AMPA into the MoTN. (C) indicates the area under curve value (AUC) value of extracellular L-glutamate level (nmol) before (basal extracellular L-glutamate level) and during perfusion with AMPA (from 20 to 180 min) of Figure 5A and 5B. Gray columns in Figure 5C indicate the AUC values of basal extracellular levels of L-glutamate in Figure 5A,B (during −60 to 0 min). **P < 0.01; relative to wild-type, @P < 0.05, @@P < 0.01; relative to control, ##P < 0.01; relative to AMPA+RJR by multivariate analysis of variance (MANOVA) with Tukey’s post hoc test.
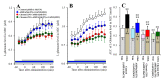
Interaction between local administration of GAP19 (Cx43 inhibitor) into the M2C and subchronic administration of therapeutic-relevant dose of ZNS (40 mg/kg/day for 7 days) on AMPA-evoked L-glutamate release in the M2C of wild-type (A) and S286L-TG (B). Rats were subchronically administered a therapeutic-relevant dose of ZNS (chronic ZNS or Chronic ZNS+GAP19) or ZNS free (control or AMPA + GAP19) using a subcutaneous osmotic pump. Perfusion medium in the M2C was commenced with MRS containing with (AMPA+GAP19 and chronic ZNS+GAP19) or without (control and chronic ZNS) 100 μM GAP19. After the stabilization of L-glutamate level in the M2C, the perfusion medium in the MoTN was switched from MRS to MRS containing 100 μM AMPA for 180 min. Ordinates indicate mean extracellular L-glutamate level (μM) (N = 6), and abscissas indicate time after AMPA-evoked stimulation (min). Blue bars indicate the perfusion with 100 μM GAP19 into the M2C. Gray bars indicate the perfusion with 100 μM AMPA into the MoTN. (C) indicates the AUC value of extracellular L-glutamate level (nmol) before (basal extracellular L-glutamate level) and during perfusion with AMPA (from 20 to 180 min) of Figure 6A,B. Gray columns in Figure 6C indicate the AUC values of basal extracellular levels of L-glutamate in Figure 6A,B. **P < 0.01; relative to wild-type, @P < 0.05, @@P < 0.01; relative to control by MANOVA with Tukey’s post hoc test.
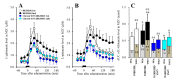
Effects of subchronic administration of therapeutic-relevant dose of ZNS (40 mg/kg/day for 7 days) on repetitive potassium-evoked L-glutamate release in the M2C of wild-type (A) and S286L-TG (B). Rats were subchronically administered with therapeutic-relevant dose of ZNS or ZNS free. Perfusion medium in the M2C was commenced with MRS. After the stabilization of L-glutamate level in the M2C, the perfusion medium was switched from MRS to HKMRS for 20 min (1st potassium-evoked stimulation) (ZNS non-treated with 1st stimulation: opened circles, ZNS administered with 1st stimulation: red circles). After the 1st potassium-evoked stimulation, the perfusate was returned to MRS for 240 min (recovery). Following recovery, the perfusate was switched to HKMRS for 20 min again (2nd potassium-evoked stimulation). After the 2nd potassium-evoked stimulation, perfusate was returned to MRS. Ordinates indicate mean extracellular L-glutamate level (μM) (N = 6), and abscissas indicate time after 1st or 2nd potassium-evoked stimulations (min). Black bars indicate the perfusion with HKMRS (1st and 2nd potassium-evoked stimulation). (C) indicates the AUC value of extracellular L-glutamate level (nmol) before (basal extracellular L-glutamate level) and after potassium-evoked stimulation (from 20 to 180 min) of Figure 7A,B. Gray columns in Figure 7C indicate the AUC values of basal extracellular levels of L-glutamate in Figure 7A,B. *P < 0.05, **P < 0.01; relative to wild-type, @P < 0.05, @@P < 0.01; relative to HKMRS (1st), $$P < 0.01 relative to HKMRS (2nd) by MANOVA with Tukey’s post hoc test.
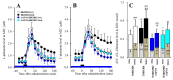
Effects of local administration of GAP19 (Cx43 inhibitor) into the M2C on repetitive potassium-evoked L-glutamate release in the M2C of wild-type (A) and S286L-TG (B). Perfusion medium in the M2C was commenced with MRS containing with or without 100 μM GAP19. After the stabilization of L-glutamate level in the M2C, the perfusion medium was switched from MRS to HKMRS containing the same agent for 20 min (1st potassium-evoked stimulation) (GAP19 non-treated with 1st stimulation: opened circles, GAP19 administered with 1st stimulation: red circles). After the 1st potassium-evoked stimulation, the perfusate was returned to MRS containing the same agent for 240 min (recovery). Following recovery, the perfusate was switched to HKMRS containing the same agent for 20 min again (2nd potassium-evoked stimulation). After the 2nd potassium-evoked stimulation, perfusate was returned to MRS containing the same agent (GAP19 non-treated with 2nd stimulation: blue circles, GAP19 administered with 2nd stimulation: green circles). Ordinates indicate mean extracellular L-glutamate level (μM) (N = 6), and abscissas indicate time after 1st or 2nd potassium-evoked stimulations (min). Black bars indicate the perfusion with HKMRS (1st and 2nd potassium-evoked stimulation). Blue bars indicate the perfusion with 100 μM GAP19 into the M2C. (C) indicates the AUC value of extracellular L-glutamate level (nmol) before (basal extracellular L-glutamate level) and after potassium-evoked stimulation (from 20 to 180 min) of Figure 8A,B. Gray columns in Figure 8C indicate the AUC values of basal extracellular levels of L-glutamate in Figure 8A,B. * P < 0.05, ** P < 0.01; relative to wild-type, @P < 0.05, @@ P < 0.01; relative to HKMRS (1st) and $ P < 0.05, $$ P < 0.01 relative to HKMRS (2nd) by MANOVA with Tukey’s post hoc test. Control data (HKMRS1 and HKMRS2) were same data of Study_3-1 (Figure 7).
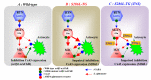
Proposed hypothesis of pathomechanisms and pathophysiology of S286L-TG. Proposed hypothesis for functional abnormalities of glutamatergic transmission in the thalamocortical pathways in wild-type (panel A), S286L-TG (panel B) and pathophysiology of ZNS-sensitive ADSHE seizure (panel C). RTN mainly projects GABAergic terminals to MoTN. Activation of α4β2-nAChR in the RTN enhances GABAergic inhibition in the RTN-MoTN pathways of wild-type (panel A). MoTN project glutamatergic terminals to the M2C. In the MoTN, both α4β2-nAChR and AMPA/glutamate receptor activate glutamatergic transmission to the M2C (panel A). Wild-type α4β2-nAChR suppresses the Cx43 expression in plasma membrane (panel A). Contrary to wild-type, loss-of-function S286L-mutant α4β2-nAChR attenuates the inhibition of Cx43 expression in the plasma membrane (panel B). Additionally, loss-of-function of S286L-mutant α4β2-nAChRs in RTN generates GABAergic disinhibition in the MoTN, leading to enhancement of glutamatergic transmission in M2C (panel B). Enhanced glutamatergic transmission in the M2C stimulates both glutamatergic pyramidal neurons and astrocytes resulting in activation of Cx43 (panel B). A combination of enhanced glutamatergic transmission and Cx43 expression in the M2C are candidate mechanisms of focus generation in the M2C (panel B). ZNS reduced Cx43 expression in the M2C (panel C). ZNS also compensates the enhanced glutamatergic transmission in the thalamocortical glutamatergic transmission via activation of II-mGluR [22] in the MoTN and GABA release [39,62] in the M2C [21].
Similar articles
-
Can rodent models elucidate the pathomechanisms of genetic epilepsy?
Okada M. Okada M. Br J Pharmacol. 2022 Apr;179(8):1620-1639. doi: 10.1111/bph.15443. Epub 2021 May 12. Br J Pharmacol. 2022. PMID: 33689168 Free PMC article. Review.
-
Fukuyama K, Okada M. Fukuyama K, et al. Int J Mol Sci. 2020 Oct 30;21(21):8142. doi: 10.3390/ijms21218142. Int J Mol Sci. 2020. PMID: 33143372 Free PMC article.
-
Fukuyama K, Fukuzawa M, Shiroyama T, Okada M. Fukuyama K, et al. Biomed Pharmacother. 2020 Jun;126:110070. doi: 10.1016/j.biopha.2020.110070. Epub 2020 Mar 10. Biomed Pharmacother. 2020. PMID: 32169758
-
Nicotinic Receptors in Sleep-Related Hypermotor Epilepsy: Pathophysiology and Pharmacology.
Becchetti A, Grandi LC, Colombo G, Meneghini S, Amadeo A. Becchetti A, et al. Brain Sci. 2020 Nov 25;10(12):907. doi: 10.3390/brainsci10120907. Brain Sci. 2020. PMID: 33255633 Free PMC article. Review.
Cited by
-
Fukuyama K, Okada M. Fukuyama K, et al. Int J Mol Sci. 2021 May 25;22(11):5623. doi: 10.3390/ijms22115623. Int J Mol Sci. 2021. PMID: 34070699 Free PMC article.
-
Can rodent models elucidate the pathomechanisms of genetic epilepsy?
Okada M. Okada M. Br J Pharmacol. 2022 Apr;179(8):1620-1639. doi: 10.1111/bph.15443. Epub 2021 May 12. Br J Pharmacol. 2022. PMID: 33689168 Free PMC article. Review.
-
Fukuyama K, Okada M. Fukuyama K, et al. Int J Mol Sci. 2022 Apr 19;23(9):4473. doi: 10.3390/ijms23094473. Int J Mol Sci. 2022. PMID: 35562864 Free PMC article.
-
Okada M, Fukuyama K, Shiroyama T, Murata M. Okada M, et al. Int J Mol Sci. 2020 Sep 24;21(19):7019. doi: 10.3390/ijms21197019. Int J Mol Sci. 2020. PMID: 32987640 Free PMC article. Review.
References
-
- Okada M., Zhu G., Yoshida S., Kaneko S. Validation criteria for genetic animal models of epilepsy. Epilepsy Seizure. 2010;3:109–120. doi: 10.3805/eands.3.109. - DOI
LinkOut - more resources
Full Text Sources
Miscellaneous