Fly motion vision is based on Reichardt detectors regardless of the signal-to-noise ratio - PubMed
- ️Thu Jan 01 2004
Fly motion vision is based on Reichardt detectors regardless of the signal-to-noise ratio
J Haag et al. Proc Natl Acad Sci U S A. 2004.
Abstract
The computational structure of an optimal motion detector was proposed to depend on the signal-to-noise ratio (SNR) of the stimulus: At low SNR, the optimal motion detector should be a correlation or "Reichardt" type, whereas at high SNR, the detector would employ a gradient scheme [Potters, M. & Bialek, W. (1994) J. Physiol. (Paris) 4, 1755-1775]. Although a large body of experiments supports the Reichardt detector as the processing scheme leading to direction selectivity in fly motion vision, in most of these studies the SNR was rather low. We therefore reinvestigated the question over a much larger SNR range. Using 2-photon microscopy, we found that local dendritic [Ca(2+)] modulations, which are characteristic of Reichardt detectors, occur in response to drifting gratings over a wide range of luminance levels and contrasts. We also explored, as another fingerprint of Reichardt detectors, the dependence of the velocity optimum on the pattern wavelength. Again, we found Reichardt-typical behavior throughout the whole luminance and contrast range tested. Our results, therefore, provide strong evidence that only a single elementary processing scheme is used in fly motion vision.
Figures
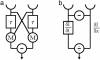
Two competing mechanisms proposed to underlie direction selectivity in fly motion detection. (a) The Reichardt detector consists of two mirror-symmetrical subunits. In each subunit, the luminance values as measured in two adjacent image locations become multiplied (M) with each other after one of them is delayed by a low-pass filter with time-constant τ. The resulting output signals of the multipliers become finally subtracted. (b) In the gradient detector, the temporal luminance gradient as measured after one photoreceptor (δI/δt, Left) is divided by the spatial luminance gradient (δI/δx). Here, the spatial gradient is approximated by the difference between the luminance values in two adjacent image locations. According to the central hypothesis being tested in this study, the mechanism should switch over from a to b with an increasing SNR in the stimulus.
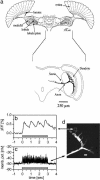
Sample recording from a motion-sensitive neuron in the lobula plate (VS2 cell). (a) Schematic outline of the fly's head ganglion (Upper, horizontal view; Lower, enlarged frontal view). Visual information from the eyes is processed retinotopically in three successive layers called the lamina, the medulla, and the lobula complex, the latter being divided into an anterior lobula and a posterior lobula plate. In the lobula plate, large tangential cells spatially integrate the output of local motion-sensitive elements. As an example, one of these cells, a VS2 cell, is depicted here. Note that information flows from the dendrite to the axon bypassing the soma which is connected to the axon only through a thin, cell-body fiber. (b) Fluorescence signal is shown during motion along the preferred direction of the cell. Fluorescence was stimulated at 850 nm. Measurements were taken from the VS2 cell shown in d within the indicated area (red box). (c) Membrane potential is recorded simultaneously from the axon of the cell (indicated by the arrow). Unlike the local dendritic signal, no modulation is visible because of spatial integration over many periodic signals, which are phase-shifted with respect to each other in different dendritic areas. (d) Projected 2-photon image stack (“extended focus”). (Scale bar: 20 μm.) Stimulus parameters: mean luminance of 50,000 cd/m2, contrast of 98%, spatial pattern wavelength of 8.3°, stimulus velocity of 10.8°/s, and temporal frequency of 1.3 Hz.
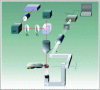
Schematic drawing of the setup used for 2-photon [Ca2+] imaging and visual stimulation in vivo. The fly is shown under an objective lens, looking down into an eyepiece mounted on a swivel arm that can be revolved around two axes (dashed lines). From the top, the femtosecond laser pulse excites the fluorophore in the visual interneuron with the emitted photons being collected by a photomultiplier. On the right side, the stimulus light path is shown: A moving grating is generated by a computer and fed through a dove prism into the optics leading to the eyepiece. SB, scan box; SL, scan lens; TL, tube lens; PMT, photomultiplier tube.
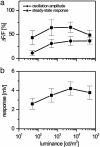
Luminance dependence of motion responses. (a) Local [Ca2+] signals of VS and HS cells. The response consists of a steady-state elevation of the [Ca2+] signal superimposed by temporal oscillations. The two signal parts are plotted independently as a function of the mean stimulus luminance. (b) Average membrane depolarization during preferred direction motion is shown as a function of mean stimulus luminance. Values represent the mean ± SEM from measurements on 11 different flies.
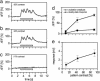
Contrast dependence of motion responses. (a-c) Examples of the local [Ca2+] response of a VS cell during preferred direction motion using three different stimulus contrasts. (d) Oscillation amplitude and steady-state [Ca2+] signal as a function of stimulus contrast. (e) Average membrane depolarization during preferred direction motion is shown as a function of stimulus contrast. Values represent the mean ± SEM from measurements on four different flies.
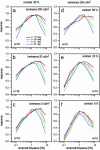
Temporal frequency tuning of the H1 neuron for different grating wavelengths λ measured at different luminance levels (a-c) and pattern contrasts (d-f). In the experiments shown in a-c, the pattern contrast was 89%. In the experiments shown in d-f, the pattern luminance was 200 cd/m2. At each luminance and contrast level, the optimum temporal frequency is clearly seen to remain constant, irrespective of the spatial wavelength of the grating. Values represent the mean ± SEM from measurements on 18 (a-c) and 15 (d-f) different flies. For each fly and each luminance or contrast condition, responses were normalized to the maximum response.
Similar articles
-
Correlation versus gradient type motion detectors: the pros and cons.
Borst A. Borst A. Philos Trans R Soc Lond B Biol Sci. 2007 Mar 29;362(1479):369-74. doi: 10.1098/rstb.2006.1964. Philos Trans R Soc Lond B Biol Sci. 2007. PMID: 17255025 Free PMC article.
-
Kondoh Y, Hasegawa Y, Okuma J, Takahashi F. Kondoh Y, et al. J Neurophysiol. 1995 Dec;74(6):2665-84. doi: 10.1152/jn.1995.74.6.2665. J Neurophysiol. 1995. PMID: 8747223
-
Propagation of photon noise and information transfer in visual motion detection.
Shi L, Borst A. Shi L, et al. J Comput Neurosci. 2006 Apr;20(2):167-78. doi: 10.1007/s10827-005-5906-3. Epub 2006 Apr 22. J Comput Neurosci. 2006. PMID: 16699840
-
Barbur JL. Barbur JL. Prog Brain Res. 2004;144:243-59. doi: 10.1016/S0079-6123(03)14417-2. Prog Brain Res. 2004. PMID: 14650853 Review.
-
A look into the cockpit of the fly: visual orientation, algorithms, and identified neurons.
Egelhaaf M, Borst A. Egelhaaf M, et al. J Neurosci. 1993 Nov;13(11):4563-74. doi: 10.1523/JNEUROSCI.13-11-04563.1993. J Neurosci. 1993. PMID: 8229185 Free PMC article. Review. No abstract available.
Cited by
-
Visual motion detection and habitat preference in Anolis lizards.
Steinberg DS, Leal M. Steinberg DS, et al. J Comp Physiol A Neuroethol Sens Neural Behav Physiol. 2016 Nov;202(11):783-790. doi: 10.1007/s00359-016-1120-1. Epub 2016 Aug 24. J Comp Physiol A Neuroethol Sens Neural Behav Physiol. 2016. PMID: 27558791
-
Local and global motion preferences in descending neurons of the fly.
Wertz A, Haag J, Borst A. Wertz A, et al. J Comp Physiol A Neuroethol Sens Neural Behav Physiol. 2009 Dec;195(12):1107-20. doi: 10.1007/s00359-009-0481-0. Epub 2009 Oct 15. J Comp Physiol A Neuroethol Sens Neural Behav Physiol. 2009. PMID: 19830435 Free PMC article.
-
Revealing nonlinear neural decoding by analyzing choices.
Yang Q, Walker E, Cotton RJ, Tolias AS, Pitkow X. Yang Q, et al. Nat Commun. 2021 Nov 16;12(1):6557. doi: 10.1038/s41467-021-26793-9. Nat Commun. 2021. PMID: 34785652 Free PMC article.
-
Predicting individual neuron responses with anatomically constrained task optimization.
Mano O, Creamer MS, Badwan BA, Clark DA. Mano O, et al. Curr Biol. 2021 Sep 27;31(18):4062-4075.e4. doi: 10.1016/j.cub.2021.06.090. Epub 2021 Jul 28. Curr Biol. 2021. PMID: 34324832 Free PMC article.
-
Near-optimal decoding of transient stimuli from coupled neuronal subpopulations.
Trousdale J, Carroll SR, Gabbiani F, Josić K. Trousdale J, et al. J Neurosci. 2014 Sep 3;34(36):12206-22. doi: 10.1523/JNEUROSCI.2671-13.2014. J Neurosci. 2014. PMID: 25186763 Free PMC article.
References
-
- Hassenstein, B. & Reichardt, W. (1956) Z. Naturforsch. 11b, 513-524.
-
- Reichardt, W. (1961) in Sensory Communication, ed. Rosenblith, W. A. (MIT Press/Wiley, New York), pp. 303-317.
-
- Reichardt, W. (1987) J. Comp. Physiol. A 161, 533-547. - PubMed
-
- Borst, A. & Egelhaaf, M. (1989) Trends Neurosci. 12, 297-306. - PubMed
-
- Egelhaaf, M. & Reichardt, W. (1987) Biol. Cybern. 56, 69-87.
Publication types
MeSH terms
LinkOut - more resources
Full Text Sources
Other Literature Sources
Miscellaneous