Second harmonic generation confocal microscopy of collagen type I from rat tendon cryosections - PubMed
- ️Sun Jan 01 2006
Second harmonic generation confocal microscopy of collagen type I from rat tendon cryosections
Theodossis A Theodossiou et al. Biophys J. 2006.
Abstract
We performed second harmonic generation (SHG) imaging of collagen in rat-tendon cryosections, using femtosecond laser scanning confocal microscopy, both in backscattering and transmission geometries. SHG transmission images of collagen fibers were spatially resolved due to a coherent, directional SHG component. This effect was enhanced with the use of an index-matching fluid (n(i) = 1.52). The average SHG intensity oscillated with wavelength in the backscattered geometry (isotropic SHG component), whereas the spectral profile was consistent with quasi-phase-matching conditions in transmission geometry (forward propagating, coherent SHG component) around 440 nm (lambda(p) = 880 nm). Collagen type I from bovine Achilles tendon was imaged for SHG in the backscattered geometry and its first-order effective nonlinear coefficient was determined (|d(eff)| approximately 0.085(+/-0.025)x10(-12)mV(-1)) by comparison to samples of inorganic materials with known effective nonlinear coefficients (LiNbO3 and LiIO3). The SHG spectral response of collagen type I from bovine Achilles tendon matched that of the rat-tendon cryosections in backscattered geometry. Collagen types I, II, and VI powders (nonfibrous) did not show any detectable SHG, indicating a lack of noncentrosymmetric crystalline structure at the molecular level. The various stages of collagen thermal denaturation were investigated in rat-tendon cryosections using SHG and bright-field imaging. Thermal denaturation resulted in the gradual destruction of the SHG signal.
Figures
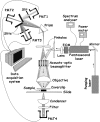
Experimental setup.
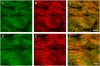
SHG imaging of collagen in 20 μm rat-foot flexor tendon cryosections mounted in PBS. The pumping wavelength was 880 nm and the SHG in backscatter geometry was imaged between 435 and 445 nm. (A) SHG in backscattering geometry (3× zoom). (B) SHG collected in transmission geometry (3× zoom). (C) Two-color overlay of images A and B (scale bar, 20 μm). Images D–F are the corresponding images for a 5× zoom (scale bar, 10 μm).
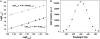
(A) Log-log plot of the SHG intensity derived from SHG imaging of rat-tendon cryosections versus pumping intensity (λp = 880 nm). Solid spheres and solid line correspond to SHG data acquired in the backscatter geometry, whereas open squares and dashed line represent the SHG data registered in transmission geometry. (B) Emission λ-scan of the SHG signal (λp = 880 nm) acquired from SHG imaging on rat-tendon cryosections. The dotted line represents a Gaussian fit. The full width at half-maximum of the fitted curve bears a 1/√2 relation to the spectral profile of the corresponding beam.
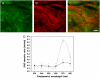
SHG imaging (λp = 880 nm) of collagen in rat-tendon cryosections mounted in index matching fluid (ni = 1.52). (A) Backscatter geometry (SHG acquisition between 435 and 445 nm). (B) Transmission geometry (through the LS-500 filter). (C) Two-color rendition of the overlay of A and B (5× zoom, scale bar, 10 μm). (D) SHG intensity ratio with/without index matching fluid versus wavelength. The solid curve represents backscatter geometry data, whereas the dashed curve represents transmission geometry data.
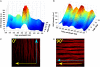
Wavelength response of the SHG intensity in rat-tendon cryosections (λp,880 nm) for various fibril orientation angles (0°, 22°, 45°, 67°, and 90°). (A) Transmission geometry. (B) Backscatter geometry. (C) SHG image (transmission) of 0° collagen-fibril long-axis orientation. (D) Same field as in C rotated by 90°. The yellow arrows in C and D represent the laser polarization. Cyan arrows denote the collagen-fibril longitudinal axis. Although the intensity scales in A and B appear comparable, the intensity in transmission geometry (A) is ∼50 times higher due to different PMT settings. The power calibration after the microscope objective for the data in Fig. 5 was performed with a wavelength-corrected Coherent (Santa Clara, CA) OP2-VIS power meter.
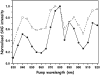
Wavelength response of the normalized SHG in collagen type I from bovine Achilles tendon (solid curve with solid spheres). The SHG spectral response of rat-tendon cryosection collagen is also shown (dashed curve with open squares) for comparison. Both SHG signals were derived in the backscattered geometry (λp = 830–910 nm). The power calibration after the microscope objective for the data in Fig. 6 was performed with a wavelength-corrected Coherent OP2-VIS power meter.
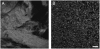
SHG images of (A) collagen type I from bovine Achilles tendon and (B) lithium niobate powder (scale bar, 40 μm). Both images were acquired between 435 and 445 nm in the backscattered geometry (λp = 880 nm).
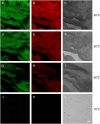
SHG imaging (λp = 880 nm) of collagen thermal denaturation in rat-tendon cryosections and the corresponding bright-field images (C, F, I, and L) (λ = 488 nm). The SHG images in backscatter geometry (A, D, G, and J) were collected in the spectral bandwidth 435–445 nm. Corresponding SHG transmission images (B, E, H, and K) were collected via the 500-nm short-pass filter. Beginning at top, rows of images (backscatter SHG, transmission SHG, and bright-field, respectively) correspond to native collagen at 25°C (A–C) and 50°C (D–F), significant thermal denaturation of collagen at 60°C (G–I), and, finally, denatured collagen at 70°C (J–L) (scale bar, 40 μm).
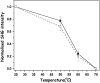
SHG intensity versus temperature as derived from the images in Fig. 8. Solid spheres and solid line represent the SHG data collected in backscatter geometry, whereas open squares and dashed line represent SHG data collected in transmission geometry.
Similar articles
-
The role of backscattering in SHG tissue imaging.
Légaré F, Pfeffer C, Olsen BR. Légaré F, et al. Biophys J. 2007 Aug 15;93(4):1312-20. doi: 10.1529/biophysj.106.100586. Epub 2007 Apr 20. Biophys J. 2007. PMID: 17449666 Free PMC article.
-
Sun Y, Chen WL, Lin SJ, Jee SH, Chen YF, Lin LC, So PT, Dong CY. Sun Y, et al. Biophys J. 2006 Oct 1;91(7):2620-5. doi: 10.1529/biophysj.106.085902. Epub 2006 Jul 7. Biophys J. 2006. PMID: 16829565 Free PMC article.
-
Polarization-dependent optical second-harmonic imaging of a rat-tail tendon.
Stoller P, Kim BM, Rubenchik AM, Reiser KM, Da Silva LB. Stoller P, et al. J Biomed Opt. 2002 Apr;7(2):205-14. doi: 10.1117/1.1431967. J Biomed Opt. 2002. PMID: 11966305
-
Form birefringence as applied to biopolymer and inorganic material supraorganization.
Vidal BC. Vidal BC. Biotech Histochem. 2010 Dec;85(6):365-78. doi: 10.3109/10520290903430496. Epub 2010 Jan 8. Biotech Histochem. 2010. PMID: 20055732 Review.
-
Bianchini P, Diaspro A. Bianchini P, et al. J Biophotonics. 2008 Dec;1(6):443-50. doi: 10.1002/jbio.200810060. J Biophotonics. 2008. PMID: 19343670 Review.
Cited by
-
Imaging the Nonlinear Susceptibility Tensor of Collagen by Nonlinear Optical Stokes Ellipsometry.
Dow XY, DeWalt EL, Sullivan SZ, Schmitt PD, Ulcickas JRW, Simpson GJ. Dow XY, et al. Biophys J. 2016 Oct 4;111(7):1361-1374. doi: 10.1016/j.bpj.2016.05.055. Biophys J. 2016. PMID: 27705760 Free PMC article.
-
Optical delineation of human malignant melanoma using second harmonic imaging of collagen.
Thrasivoulou C, Virich G, Krenacs T, Korom I, Becker DL. Thrasivoulou C, et al. Biomed Opt Express. 2011 Apr 20;2(5):1282-95. doi: 10.1364/BOE.2.001282. Biomed Opt Express. 2011. PMID: 21559140 Free PMC article.
-
Circular Dichroism Spectroscopy of Collagen Fibrillogenesis: A New Use for an Old Technique.
Drzewiecki KE, Grisham DR, Parmar AS, Nanda V, Shreiber DI. Drzewiecki KE, et al. Biophys J. 2016 Dec 6;111(11):2377-2386. doi: 10.1016/j.bpj.2016.10.023. Biophys J. 2016. PMID: 27926839 Free PMC article.
-
Niklason LE, Yeh AT, Calle EA, Bai Y, Valentín A, Humphrey JD. Niklason LE, et al. Proc Natl Acad Sci U S A. 2010 Feb 23;107(8):3335-9. doi: 10.1073/pnas.0907813106. Epub 2009 Dec 1. Proc Natl Acad Sci U S A. 2010. PMID: 19955446 Free PMC article.
-
The structural origin of second harmonic generation in fascia.
Rivard M, Laliberté M, Bertrand-Grenier A, Harnagea C, Pfeffer CP, Vallières M, St-Pierre Y, Pignolet A, El Khakani MA, Légaré F. Rivard M, et al. Biomed Opt Express. 2010 Dec 1;2(1):26-36. doi: 10.1364/BOE.2.000026. Biomed Opt Express. 2010. PMID: 21326632 Free PMC article.
References
-
- Franken, P. A., A. E. Hill, C. W. Peters, and G. Weinreich. 1961. Generation of optical harmonics. Phys. Rev. Lett. 7:118–119.
-
- Bloembergen, N. 1996. Nonlinear Optics. World Scientific Publishing, Hackensack, NJ.
-
- Zernike, F., and J. E. Midwinter. 1973. Applied Nonlinear Optics. Wiley, New York.
-
- Shen, Y. R. 1989. The Principles of Nonlinear Optics. Wiley, New York.
-
- Yariv, A. 1991. Optical Electronics. Saunders College Publishing, Fort Worth, TX.
MeSH terms
Substances
LinkOut - more resources
Full Text Sources
Other Literature Sources