Visual map development: bidirectional signaling, bifunctional guidance molecules, and competition - PubMed
Review
Visual map development: bidirectional signaling, bifunctional guidance molecules, and competition
David A Feldheim et al. Cold Spring Harb Perspect Biol. 2010 Nov.
Abstract
Topographic maps are a two-dimensional representation of one neural structure within another and serve as the main strategy to organize sensory information. The retina's projection via axons of retinal ganglion cells to midbrain visual centers, the optic tectum/superior colliculus, is the leading model to elucidate mechanisms of topographic map formation. Each axis of the retina is mapped independently using different mechanisms and sets of axon guidance molecules expressed in gradients to achieve the goal of representing a point in the retina onto a point within the target. An axon's termination along the temporal-nasal mapping axis is determined by opposing gradients of EphAs and ephrin-As that act through their forward and reverse signaling, respectively, within the projecting axons, each of which inhibits interstitial branching, cooperating with a branch-promoting activity, to generate topographic specific branching along the shaft of the parent axons that overshoot their correct termination zone along the anterior-posterior axis of the target. The dorsal-ventral termination position is then determined using a gradient of ephrin-B that can act as a repellent or attractant depending on the ephrin-B concentration relative to EphB levels on the interstitial branches to guide them along the medial-lateral axis of the target to their correct termination zone, where they arborize. In both cases, axon-axon competition results in axon mapping based on relative rather than absolute levels of repellent or attractant activity. The map is subsequently refined through large-scale pruning driven in large part by patterned retinal activity.
Figures
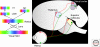
Visual areas in the brain are mapped topographically. In the mammalian visual system, photoreceptors in the retina detect photons and transfers this information to multiple visual areas in the brain, including the superior colliculus (SC) in the midbrain and the dorsal lateral geniculate nucleus (dLGN) in the thalamus. The dLGN then transfers this information to the primary visual cortex (V1). Each of the projections is mapped topographically such that neighboring inputs in the visual field (shown as different colors) maintain their relationships as information is transferred, creating a visuotopic map in each processing area.
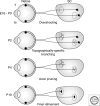
Developmental stages in mapping temporal-nasal retinal axis on anterior-posterior axis of colliculus/tectum in mice and chicks. Initially RGC axons enter the SC at the anterior end and project to the posterior SC overshooting their topographically appropriate termination zone (dashed circles). Then branching occurs from the axon at the topographically specific location followed by pruning of the posterior axon. At later stages the branches are stabilized, and refine to form strong synapses. In mice this process starts late in embryogenesis and lasts through the first week of life (E, embryonic day post conception; P, postnatal).
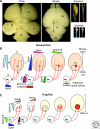
Substantial size differences in midbrain targets and distinct mechanisms of map development exhibited by model systems. (A) Photographs are of the primary model systems currently used to study retinocollicular/retinotectal map development, taken at critical ages during map formation. Photos are at the same scale. Photos of chick and mouse show dorsal surface of brain. The white dashed line on the chick optic tectum (ot) indicates the anterior (A)–posterior (P) axis. The bracket on the mouse midbrain shows the extent of the AP axis of the superior colliculus (SC); the extent of the Lateral (L)–Medial (M) axis is marked by L and M. Paired photos for Xenopus (frog) and zebrafish show lateral (left) and dorsal (right) views: the AP extent of the optic tectum (OT) in each is marked by small white bars adjacent to the brain. The dramatic differences in target size between the warm-blooded vertebrates (chick and mouse) relative to the cold-blooded vertebrates (frog and fish) may underlie the significant differences in mechanisms employed by RGC axons to develop retinotopic maps in them. (B and C) Mechanisms and molecules controlling retinotopic mapping in (B) mouse and chick and (C) frog and zebrafish. The names and distributions of molecules and activities shown in vivo to control the mapping mechanisms exhibited at each stage are indicated. The gradients may represent the consensus distribution for a combination of related molecules (e.g., EphAs), which are not listed individually because of distinctions in the individual members expressed and the precise distributions between species. Molecules and activities other than those listed are likely to participate in topographic mapping. (See facing page for legend.) (B) In mouse and chick RGC axons enter the OT/SC over a broad LM extent and significantly overshoot their future termination zone (TZ, circle). Interstitial branches form de novo from the axon shaft around the correct anterior–posterior (A–P) position of their future TZ. Branches are directed along the lateral–medial (L–M) axis of the OT/SC toward the position of the future TZ, in which they arborize in a domain encompassing the forming TZ. The broad, loose array of arbors is refined to a dense TZ in the topographically appropriate location. (C) In frogs and fish the RGC growth cone extends to the TZ in which it forms a terminal arbor through a process of terminal branching of the growth cone and backbranching immediately behind the growth cone. The tectum expands as terminal arborizations elaborate and refine into a mature TZ. See text for further description, function of topographic guidance molecules and patterned activity, and references. cb, cerebellum; ctx, cortex; D, dorsal; fb, forebrain; hb, hindbrain; N, nasal; T, temporal; V, ventral. (Modified from McLaughlin et al. 2003c [© Elsevier].)
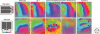
Functional imaging of adult SC in wild type and mutant mice. Top panels show that the D-V retinal projection onto the medial-lateral axis of the SC is largely normal in the mice indicated. Bottom panels show that the N-T retinal projections map onto the anterior–posterior axis of the SC. Note that ephrin-A tkos have a patchy map (arrows highlight red regions [nasal axon mapping regions] that are noncontiguous), whereas ephrin-A2/A5/β2 tkos have no map, specifically along the N-T mapping axis. Amazingly, the EphA3-ki mouse maps are duplicated within the SC (arrows mark regions of duplication). (Adapted from Cang et al. 2005b, ; Triplett et al. 2009.)
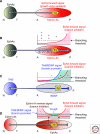
Regulation of topographic-specific interstitial branching along anterior–posterior axis of tectum/colliculus: the critical determinant of retinotopic mapping. Shown are models based on known expression patterns of EphAs, ephrin-As, TrkB, and BDNF, their influences on RGC axons, and their proposed roles or deficiencies in development of topographic maps. All models have as recurring theme the graded repellent activity that preferentially affects temporal RGC axons and is achieved by EphA forward signaling. (A) EphA is expressed in a high to low temporal (T) to nasal (N) gradient by RGCs, and ephrin—As in a low to high anterior (A) to posterior (P) gradient in the optic tectum (OT)/superior colliculus (SC). This receptor-legend pairing generates through EphA forward signaling a high to low AP gradient of repellent activity that preferential affects temporal RGC axons, and is in principle sufficient to topographically guide RGC axonal growth cones along the AP axis to their appropriate terminal zone (TZ). This mapping mechanism is adequate in lower vertebrates. (B) However, a single repulsive gradient cannot generate the topographic interstitial branching of RGC axons along the AP axis observed in chicks and rodents, because branching would occur at a greater frequency more anterior to the TZ. This is not observed in vivo—branching is focused on the AP position of the TZ and is lower both anterior and posterior it. (C) In principal, topographic-specific interstitial branching could be achieved by a model with a gradient of molecules with branch promoting activities that cooperate with the EphA forward signaling inhibition of branching. (See facing page for legend.) Branching is prevented posterior to the correct TZ by ephrin-A repellent activity mediated by EphA forward signaling, and does not occur anterior to the correct TZ because the branch promoting activity is subthreshold. Branching occurs at the AP position in which the branch promoter is suprathreshold and the branch inhibitor is subthreshold. TrkB, in a similar distribution to EphAs in the retina (and/or, if TrkB were graded along each RGC axon), and BDNF in the OT/SC have the appropriate activities to act as the graded branch promoter, and cooperate with the graded branch inhibitor generated by EphA/ephrin-A forward signaling. (D) Depicted is the favored model to generate topographic-specific interstitial branching in the chick OT and mouse SC and is based on known activities (see text). Topographic-specific branching is generated by opposing gradients of branch inhibiting molecules combined with a branch promoting activity. In this model, the repellent gradient because of EphA/ephrin-A forward signaling inhibits branching posterior to the TZ and the repellent gradient because ephrin-A/EphA reverse signaling inhibits branching anterior to the TZ. Branching occurs preferentially at a trough in the opposing repellent gradients that is subthreshold for branch inhibition and is located at the correct AP position of the TZ. At the position of this trough, the activity of a branch promoter (such as BDNF signaling through TrkB) is suprathreshold for branch inhibition and generates branching at this topographically correct location for the TZ. The distributions of EphAs, ephrin-As, BDNF, and trkB in the retina and OT/SC, are appropriate for this model, and it is consistent with gain- and loss-of-function analyses of map development in chicks and mice. See Text for further description and references. (Modified from McLaughlin and O’Leary 2005 [© Annual Reviews].)
Similar articles
-
O'Leary DD, McLaughlin T. O'Leary DD, et al. Prog Brain Res. 2005;147:43-65. doi: 10.1016/S0079-6123(04)47005-8. Prog Brain Res. 2005. PMID: 15581697 Review.
-
Yates PA, Holub AD, McLaughlin T, Sejnowski TJ, O'Leary DD. Yates PA, et al. J Neurobiol. 2004 Apr;59(1):95-113. doi: 10.1002/neu.10341. J Neurobiol. 2004. PMID: 15007830 Free PMC article.
-
Eph and ephrin signaling in the formation of topographic maps.
Triplett JW, Feldheim DA. Triplett JW, et al. Semin Cell Dev Biol. 2012 Feb;23(1):7-15. doi: 10.1016/j.semcdb.2011.10.026. Epub 2011 Oct 24. Semin Cell Dev Biol. 2012. PMID: 22044886 Free PMC article. Review.
-
Ortalli AL, Fiore L, Di Napoli J, Rapacioli M, Salierno M, Etchenique R, Flores V, Sanchez V, Carri NG, Scicolone G. Ortalli AL, et al. PLoS One. 2012;7(6):e38566. doi: 10.1371/journal.pone.0038566. Epub 2012 Jun 7. PLoS One. 2012. PMID: 22685584 Free PMC article.
-
Yates PA, Roskies AL, McLaughlin T, O'Leary DD. Yates PA, et al. J Neurosci. 2001 Nov 1;21(21):8548-63. doi: 10.1523/JNEUROSCI.21-21-08548.2001. J Neurosci. 2001. PMID: 11606643 Free PMC article.
Cited by
-
Maiorano NA, Hindges R. Maiorano NA, et al. Nat Commun. 2013;4:1938. doi: 10.1038/ncomms2926. Nat Commun. 2013. PMID: 23733098 Free PMC article.
-
Spontaneous Afferent Activity Carves Olfactory Circuits.
Redolfi N, Lodovichi C. Redolfi N, et al. Front Cell Neurosci. 2021 Mar 9;15:637536. doi: 10.3389/fncel.2021.637536. eCollection 2021. Front Cell Neurosci. 2021. PMID: 33767612 Free PMC article.
-
Vision-Dependent and -Independent Molecular Maturation of Mouse Retinal Ganglion Cells.
Whitney IE, Butrus S, Dyer MA, Rieke F, Sanes JR, Shekhar K. Whitney IE, et al. Neuroscience. 2023 Jan 1;508:153-173. doi: 10.1016/j.neuroscience.2022.07.013. Epub 2022 Jul 21. Neuroscience. 2023. PMID: 35870562 Free PMC article.
-
Morikawa RK, Kanamori T, Yasunaga K, Emoto K. Morikawa RK, et al. Proc Natl Acad Sci U S A. 2011 Nov 29;108(48):19389-94. doi: 10.1073/pnas.1109843108. Epub 2011 Nov 14. Proc Natl Acad Sci U S A. 2011. PMID: 22084112 Free PMC article.
-
Cioni JM, Wong HH, Bressan D, Kodama L, Harris WA, Holt CE. Cioni JM, et al. Neuron. 2018 Mar 7;97(5):1078-1093.e6. doi: 10.1016/j.neuron.2018.01.027. Neuron. 2018. PMID: 29518358 Free PMC article.
References
-
- Braisted JE, McLaughlin T, Wang HU, Friedman GC, Anderson DJ, O’Leary DDM 1997. Graded and lamina-specific distributions of ligands of EphB receptor tyrosine kinases in the developing retinotectal system. Dev Biol 191: 14–28 - PubMed
-
- Brown A, Yates PA, Burrols P, Ortuno D, Vaidya A, Jessell TM, Pfaff SL, O’Leary DDM, Lemke G 2000. Topographic mapping from the retina to the midbrain is controlled by relative but not absolute levels of EphA receptor signaling. Cell 102: 77–88 - PubMed
Publication types
MeSH terms
Substances
LinkOut - more resources
Full Text Sources
Research Materials