Optogenetic reporters: Fluorescent protein-based genetically encoded indicators of signaling and metabolism in the brain - PubMed
Review
Optogenetic reporters: Fluorescent protein-based genetically encoded indicators of signaling and metabolism in the brain
Mathew Tantama et al. Prog Brain Res. 2012.
Abstract
Fluorescent protein technology has evolved to include genetically encoded biosensors that can monitor levels of ions, metabolites, and enzyme activities as well as protein conformation and even membrane voltage. They are well suited to live-cell microscopy and quantitative analysis, and they can be used in multiple imaging modes, including one- or two-photon fluorescence intensity or lifetime microscopy. Although not nearly complete, there now exists a substantial set of genetically encoded reporters that can be used to monitor many aspects of neuronal and glial biology, and these biosensors can be used to visualize synaptic transmission and activity-dependent signaling in vitro and in vivo. In this review, we present an overview of design strategies for engineering biosensors, including sensor designs using circularly permuted fluorescent proteins and using fluorescence resonance energy transfer between fluorescent proteins. We also provide examples of indicators that sense small ions (e.g., pH, chloride, zinc), metabolites (e.g., glutamate, glucose, ATP, cAMP, lipid metabolites), signaling pathways (e.g., G protein-coupled receptors, Rho GTPases), enzyme activities (e.g., protein kinase A, caspases), and reactive species. We focus on examples where these genetically encoded indicators have been applied to brain-related studies and used with live-cell fluorescence microscopy.
Copyright © 2012 Elsevier B.V. All rights reserved.
Figures
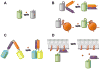
Design strategies for engineering genetically-encoded indicators. Fluorescent proteins are shown as cylinders. Sensor domains are boxes or spheres in orange or purple. Target ligands are shown in red. Examples are shown for indicators of small molecule analytes, but the designs can also be applied to engineer indicators of enzyme activity or protein activation. (A) A single environmentally-sensitive fluorescent protein acts as the sensor and reporter. (B) Circular-permutation of a fluorescent protein renders its fluorescence sensitive to a conformational change in a sensor domain. The sensor domain may consist of two proteins or peptides attached to the new termini of the circularly-permuted fluorescent protein (top). Alternatively, the circularly-permuted fluorescent protein may be inserted into a sensor protein in a region that experiences a conformation change (bottom). (C) Fluorescent proteins are attached to two parts of a sensor domain that experience a relative motion, and the conformational change can alter the distance or orientation between the fluorescent proteins, changing the FRET efficiency. (D) A fluorescent protein is attached to a sensor domain that localizes differently depending on ligand binding or activation. In the example shown, the indicator localizes to the plasma membrane or cytosol depending on the ligand status.
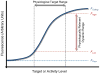
A hypothetical GEI dose-response curve. The physiologically relevant dynamic range (FHigh/FLow) is usually smaller than the GEI’s maximum fluorescence dynamic range (FCeiling/FFloor) because only a portion of the total sensing range is sampled in the physiological scenario.
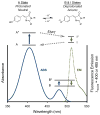
Protonation of the fluorescent protein chromophore and its effects on the absorbance and fluorescence spectra. The example is theoretical but based on the wild type green fluorescent protein chromophore and spectra. In the physiological pH range, the chromophore can be protonated in a neutral “A” state or ionized in an anionic “B” state (top). Two peaks in the absorbance spectrum (solid blue curve) reflect the two states. A single peak is observed in the fluorescence emission spectrum (dotted green curve) when exciting the B state, and a similar emission spectrum is observed when exciting the A state because excited-state proton transfer occurs, creating an anionic excited state I* very similar to the B* anionic excited state. Simplified Jablonski diagrams are superimposed to represent energy transitions following absorption (blue arrow) and resulting in fluorescence emission (green arrow). The B and B* energy levels are shown lower in the diagram for visual clarity and are not indicative of actual energy differences with the A or I states.
Similar articles
-
Live-cell imaging of cell signaling using genetically encoded fluorescent reporters.
Ni Q, Mehta S, Zhang J. Ni Q, et al. FEBS J. 2018 Jan;285(2):203-219. doi: 10.1111/febs.14134. Epub 2017 Jul 6. FEBS J. 2018. PMID: 28613457 Free PMC article. Review.
-
Zhang C, Wei ZH, Ye BC. Zhang C, et al. Biotechnol J. 2013 Nov;8(11):1280-91. doi: 10.1002/biot.201300001. Epub 2013 Sep 25. Biotechnol J. 2013. PMID: 24591186 Review.
-
Genetically Encoded Fluorescent Biosensors to Explore AMPK Signaling and Energy Metabolism.
Pelosse M, Cottet-Rousselle C, Grichine A, Berger I, Schlattner U. Pelosse M, et al. Exp Suppl. 2016;107:491-523. doi: 10.1007/978-3-319-43589-3_20. Exp Suppl. 2016. PMID: 27812993 Review.
-
Live-cell Imaging with Genetically Encoded Protein Kinase Activity Reporters.
Maryu G, Miura H, Uda Y, Komatsubara AT, Matsuda M, Aoki K. Maryu G, et al. Cell Struct Funct. 2018 Apr 25;43(1):61-74. doi: 10.1247/csf.18003. Epub 2018 Mar 17. Cell Struct Funct. 2018. PMID: 29553079 Review.
-
Schmitt DL, Mehta S, Zhang J. Schmitt DL, et al. Curr Opin Chem Biol. 2020 Feb;54:63-69. doi: 10.1016/j.cbpa.2019.11.005. Epub 2020 Jan 3. Curr Opin Chem Biol. 2020. PMID: 31911398 Free PMC article. Review.
Cited by
-
Brain/MINDS: brain-mapping project in Japan.
Okano H, Miyawaki A, Kasai K. Okano H, et al. Philos Trans R Soc Lond B Biol Sci. 2015 May 19;370(1668):20140310. doi: 10.1098/rstb.2014.0310. Philos Trans R Soc Lond B Biol Sci. 2015. PMID: 25823872 Free PMC article. Review.
-
Glucose, lactate, and shuttling of metabolites in vertebrate retinas.
Hurley JB, Lindsay KJ, Du J. Hurley JB, et al. J Neurosci Res. 2015 Jul;93(7):1079-92. doi: 10.1002/jnr.23583. Epub 2015 Mar 20. J Neurosci Res. 2015. PMID: 25801286 Free PMC article. Review.
-
Werley CA, Boccardo S, Rigamonti A, Hansson EM, Cohen AE. Werley CA, et al. Nat Commun. 2020 Aug 4;11(1):3881. doi: 10.1038/s41467-020-17607-5. Nat Commun. 2020. PMID: 32753572 Free PMC article.
-
Lu FM, Hilgemann DW. Lu FM, et al. J Gen Physiol. 2017 Jul 3;149(7):727-749. doi: 10.1085/jgp.201711780. Epub 2017 Jun 12. J Gen Physiol. 2017. PMID: 28606910 Free PMC article.
-
Culture and adenoviral infection of sinoatrial node myocytes from adult mice.
St Clair JR, Sharpe EJ, Proenza C. St Clair JR, et al. Am J Physiol Heart Circ Physiol. 2015 Aug 1;309(3):H490-8. doi: 10.1152/ajpheart.00068.2015. Epub 2015 May 22. Am J Physiol Heart Circ Physiol. 2015. PMID: 26001410 Free PMC article.
References
-
- Agbulut O, Coirault C, Niederlander N, Huet A, Vicart P, Hagege A, Puceat M, Menasche P. GFP expression in muscle cells impairs actin-myosin interactions: implications for cell therapy. Nat Methods. 2006;3:331. - PubMed
Publication types
MeSH terms
Substances
Grants and funding
LinkOut - more resources
Full Text Sources