Retrograde bilin signaling enables Chlamydomonas greening and phototrophic survival - PubMed
- ️Tue Jan 01 2013
. 2013 Feb 26;110(9):3621-6.
doi: 10.1073/pnas.1222375110. Epub 2013 Jan 23.
David Casero, Rachel M Dent, Sean Gallaher, Wenqiang Yang, Nathan C Rockwell, Shelley S Martin, Matteo Pellegrini, Krishna K Niyogi, Sabeeha S Merchant, Arthur R Grossman, J Clark Lagarias
Affiliations
- PMID: 23345435
- PMCID: PMC3587268
- DOI: 10.1073/pnas.1222375110
Retrograde bilin signaling enables Chlamydomonas greening and phototrophic survival
Deqiang Duanmu et al. Proc Natl Acad Sci U S A. 2013.
Abstract
The maintenance of functional chloroplasts in photosynthetic eukaryotes requires real-time coordination of the nuclear and plastid genomes. Tetrapyrroles play a significant role in plastid-to-nucleus retrograde signaling in plants to ensure that nuclear gene expression is attuned to the needs of the chloroplast. Well-known sites of synthesis of chlorophyll for photosynthesis, plant chloroplasts also export heme and heme-derived linear tetrapyrroles (bilins), two critical metabolites respectively required for essential cellular activities and for light sensing by phytochromes. Here we establish that Chlamydomonas reinhardtii, one of many chlorophyte species that lack phytochromes, can synthesize bilins in both plastid and cytosol compartments. Genetic analyses show that both pathways contribute to iron acquisition from extracellular heme, whereas the plastid-localized pathway is essential for light-dependent greening and phototrophic growth. Our discovery of a bilin-dependent nuclear gene network implicates a widespread use of bilins as retrograde signals in oxygenic photosynthetic species. Our studies also suggest that bilins trigger critical metabolic pathways to detoxify molecular oxygen produced by photosynthesis, thereby permitting survival and phototrophic growth during the light period.
Conflict of interest statement
The authors declare no conflict of interest.
Figures
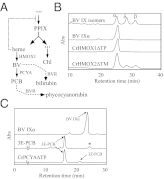
Both annotated C. reinhardtii heme oxygenases (HMOX1 and HMOX2) and PCYA bilin reductase genes encode catalytically active enzymes. (A) Tetrapyrrole pathways of C. reinhardtii and their perturbation by artificial introduction of mammalian biliverdin reductase (BVR). Feedback inhibition of tetrapyrrole synthesis by heme is indicated by a dotted line, and depletion of heme upon plastid expression of BVR is indicated by dashed lines. Chl, chlorophyll; PPIX, protoporphyrin IX. (B) HPLC elution profiles of HMOX metabolites and mixtures of BV isomers (α, δ, and β) obtained by chemical oxidative degradation of hemin. (C) HPLC analysis of PCYA-catalyzed formation of PCB from BV IXα and bilin standards (top traces). The asterisk indicates an impurity.
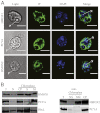
Subcellular distribution of heme oxygenases and bilin reductase. (A) Localization of HMOX1, PCYA, and HMOX2. DAPI, 4′,6-diamidino-2-phenylindole staining of the nucleus and chloroplast DNA; IF, indirect immunofluorescence; Light, bright field image; Merge, overlay of IF and DAPI. Pyrenoid (P) and nucleus (N) are shown with arrows. (Scale bars, 5 µm.) (B) Biochemical fractionation of HMOX1, PCYA,and HMOX2 as revealed by immunoblot analysis of total cellular proteins (T), nonchloroplast supernatant (N) and intact chloroplast (CP), and soluble (S) and membrane-associated (M) proteins from chloroplasts. The nonchloroplast supernatant was further separated into soluble (NS) and membrane (NM) fractions. Thirty micrograms of protein of each fraction was loaded for immunoblot.
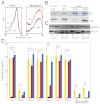
Chloroplast HMOX1 is responsible for synthesis of phycocyanobilin (PCB), and both heme oxygenases participate in heme iron assimilation in vivo. (A) Absorption spectra of native and denatured PCB-adducts of chloroplast-targeted cyanobacteriochrome NpF2164g5 purified from C. reinhardtii wild-type strain 4A+ or from PCB-producing E. coli. (B) NpF2164g5 purified from transgenic 4A+, hmox1, or E. coli cultures analyzed by Coomassie Blue (CB) staining (Upper) or by zinc-dependent fluorescence (Lower) for the presence of covalently bound PCB. Purified NpF2164g5 protein from hmox1 cells was still capable of binding PCB in vitro. (C) Immunoblot analysis of HMOX1 and PCYA in 4A+, hmox1, and two hmox1 complementing lines (ho1C1 and ho1C2) grown in suspension culture heterotrophically in darkness (D) or photoautotrophically under continuous light (L) at a fluence rate of 100 μmol photons⋅m−2⋅s−1. (D) Growth of C. reinhardtii with exogenous heme as iron source as measured by stationary-phase chlorophyll levels. All strains were grown in complete TAP medium (yellow columns), iron-free medium (black), or iron-free medium supplemented with either 10 µM hemin (red) or 20 µM Fe3+ (blue). The percent chlorophyll content of hemin-supplemented cultures relative to those grown on complete medium is shown for each genotype. Bars indicate the SD of three to four replicates.
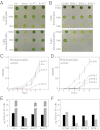
Photoautotrophic growth of both hmox1 mutant and BVR-expressing transgenic lines of C. reinhardtii is severely impaired. (A) Comparative growth on agar medium of 4A+, hmox1, and two complemented hmox1 lines (ho1C1 and ho1C2) in the dark (with acetate, heterotrophic) or under light (∼180 µmol photons⋅m−2⋅s−1, no acetate, photoautotrophic). (B) Comparative growth on agar medium of CC849 (wall-less wild type) and three BVR expression lines as in A. (C) Batch culture growth curves for hmox1 and complemented lines in continuous light (∼180 µmol photons⋅m−2⋅s−1) inoculated at the same optical density (OD800 = 0.001, ∼1.0 × 104 cells/mL). Bars indicate the SD of three to four replicates. (D) Suspension culture growth curves for the three BVR lines in continuous light as in C. (E) Normalized total chlorophyll (Chl a + Chl b) content per 107 cells in 4A+, hmox1, and complemented ho1C1 and ho1C2 cell lines. Black bars, heterotrophic (with acetate) dark-grown samples; gray bars, photoautotrophic (no acetate) light-grown samples. (F) Normalized total chlorophyll in BVR lines as in E.
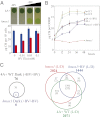
Biliverdin rescues the light-sensitive and chlorophyll-deficient phenotypes of the hmox1 mutant. (A) Photoautotrophic (∼180 µmol photons⋅m−2⋅s−1) growth on agar media (Upper) and normalized total chlorophyll levels (Lower) of 4A+ and hmox1 suspension cultures in the presence of BV IXα. Normalized total chlorophyll levels of 4A+ (blue) and hmox1 (red) after 7 d of growth is presented as described in Fig. 4. (B) Time course of chlorophyll accumulation upon transfer to light. Exponential-phase cultures grown in TAP medium in darkness supplemented with or without 0.1 mM BV IXα were transferred to white light (∼180 µmol photons⋅m−2⋅s−1). Bars indicate the SD of three replicates. (C) RNA-seq reveals that BV regulates gene expression in darkness (Left) and also rescues a subset of light-regulated C. reinhardtii genes following the transfer from dark to light (Right). The diagrams show the number of nonoverlapping and shared differentially regulated transcripts with ≥twofold change and false discovery rate < 1% for each pairwise comparison. Numbers of genes that are also BV-regulated in the dark are shown in parentheses (Right). Genes of each subgroup are listed in
Datasets S1and
S2.
Comment in
-
Surprising roles for bilins in a green alga.
Rochaix JD. Rochaix JD. Proc Natl Acad Sci U S A. 2013 Feb 26;110(9):3218-9. doi: 10.1073/pnas.1300399110. Epub 2013 Feb 14. Proc Natl Acad Sci U S A. 2013. PMID: 23412335 Free PMC article. No abstract available.
Similar articles
-
Bilin-Dependent Photoacclimation in Chlamydomonas reinhardtii.
Wittkopp TM, Schmollinger S, Saroussi S, Hu W, Zhang W, Fan Q, Gallaher SD, Leonard MT, Soubeyrand E, Basset GJ, Merchant SS, Grossman AR, Duanmu D, Lagarias JC. Wittkopp TM, et al. Plant Cell. 2017 Nov;29(11):2711-2726. doi: 10.1105/tpc.17.00149. Epub 2017 Oct 30. Plant Cell. 2017. PMID: 29084873 Free PMC article.
-
Bilin-dependent regulation of chlorophyll biosynthesis by GUN4.
Zhang W, Willows RD, Deng R, Li Z, Li M, Wang Y, Guo Y, Shi W, Fan Q, Martin SS, Rockwell NC, Lagarias JC, Duanmu D. Zhang W, et al. Proc Natl Acad Sci U S A. 2021 May 18;118(20):e2104443118. doi: 10.1073/pnas.2104443118. Proc Natl Acad Sci U S A. 2021. PMID: 33975960 Free PMC article.
-
Ferredoxin-dependent bilin reductases in eukaryotic algae: Ubiquity and diversity.
Rockwell NC, Lagarias JC. Rockwell NC, et al. J Plant Physiol. 2017 Oct;217:57-67. doi: 10.1016/j.jplph.2017.05.022. Epub 2017 May 31. J Plant Physiol. 2017. PMID: 28641882 Free PMC article.
-
Function and distribution of bilin biosynthesis enzymes in photosynthetic organisms.
Dammeyer T, Frankenberg-Dinkel N. Dammeyer T, et al. Photochem Photobiol Sci. 2008 Oct;7(10):1121-30. doi: 10.1039/b807209b. Epub 2008 Jul 7. Photochem Photobiol Sci. 2008. PMID: 18846276 Review.
-
Rea G, Antonacci A, Lambreva MD, Mattoo AK. Rea G, et al. Plant Sci. 2018 Jul;272:193-206. doi: 10.1016/j.plantsci.2018.04.020. Epub 2018 Apr 27. Plant Sci. 2018. PMID: 29807591 Review.
Cited by
-
Phytochrome evolution in 3D: deletion, duplication, and diversification.
Rockwell NC, Lagarias JC. Rockwell NC, et al. New Phytol. 2020 Mar;225(6):2283-2300. doi: 10.1111/nph.16240. Epub 2019 Nov 2. New Phytol. 2020. PMID: 31595505 Free PMC article. Review.
-
Schluchter WM, Babin CH, Liu X, Bieller A, Shen G, Alvey RM, Bryant DA. Schluchter WM, et al. Microorganisms. 2023 Oct 20;11(10):2593. doi: 10.3390/microorganisms11102593. Microorganisms. 2023. PMID: 37894251 Free PMC article.
-
UV-B Perception and Acclimation in Chlamydomonas reinhardtii.
Tilbrook K, Dubois M, Crocco CD, Yin R, Chappuis R, Allorent G, Schmid-Siegert E, Goldschmidt-Clermont M, Ulm R. Tilbrook K, et al. Plant Cell. 2016 Apr;28(4):966-83. doi: 10.1105/tpc.15.00287. Epub 2016 Mar 28. Plant Cell. 2016. PMID: 27020958 Free PMC article.
-
Bilin-Dependent Photoacclimation in Chlamydomonas reinhardtii.
Wittkopp TM, Schmollinger S, Saroussi S, Hu W, Zhang W, Fan Q, Gallaher SD, Leonard MT, Soubeyrand E, Basset GJ, Merchant SS, Grossman AR, Duanmu D, Lagarias JC. Wittkopp TM, et al. Plant Cell. 2017 Nov;29(11):2711-2726. doi: 10.1105/tpc.17.00149. Epub 2017 Oct 30. Plant Cell. 2017. PMID: 29084873 Free PMC article.
-
The Red Edge: Bilin-Binding Photoreceptors as Optogenetic Tools and Fluorescence Reporters.
Tang K, Beyer HM, Zurbriggen MD, Gärtner W. Tang K, et al. Chem Rev. 2021 Dec 22;121(24):14906-14956. doi: 10.1021/acs.chemrev.1c00194. Epub 2021 Oct 20. Chem Rev. 2021. PMID: 34669383 Free PMC article. Review.
References
-
- Apel K, Hirt H. Reactive oxygen species: Metabolism, oxidative stress, and signal transduction. Annu Rev Plant Biol. 2004;55:373–399. - PubMed
-
- Christie JM. Phototropin blue-light receptors. Annu Rev Plant Biol. 2007;58:21–45. - PubMed
-
- Chaves I, et al. The cryptochromes: Blue light photoreceptors in plants and animals. Annu Rev Plant Biol. 2011;62:335–364. - PubMed
Publication types
MeSH terms
Substances
LinkOut - more resources
Full Text Sources
Other Literature Sources
Molecular Biology Databases