DNA binding properties of human Cdc45 suggest a function as molecular wedge for DNA unwinding - PubMed
DNA binding properties of human Cdc45 suggest a function as molecular wedge for DNA unwinding
Anna Szambowska et al. Nucleic Acids Res. 2014 Feb.
Abstract
The cell division cycle protein 45 (Cdc45) represents an essential replication factor that, together with the Mcm2-7 complex and the four subunits of GINS, forms the replicative DNA helicase in eukaryotes. Recombinant human Cdc45 (hCdc45) was structurally characterized and its DNA-binding properties were determined. Synchrotron radiation circular dichroism spectroscopy, dynamic light scattering, small-angle X-ray scattering and atomic force microscopy revealed that hCdc45 exists as an alpha-helical monomer and possesses a structure similar to its bacterial homolog RecJ. hCdc45 bound long (113-mer or 80-mer) single-stranded DNA fragments with a higher affinity than shorter ones (34-mer). hCdc45 displayed a preference for 3' protruding strands and bound tightly to single-strand/double-strand DNA junctions, such as those presented by Y-shaped DNA, bubbles and displacement loops, all of which appear transiently during the initiation of DNA replication. Collectively, our findings suggest that hCdc45 not only binds to but also slides on DNA with a 3'-5' polarity and, thereby acts as a molecular 'wedge' to initiate DNA strand displacement.
Figures
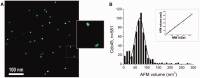
hCdc45 represents a well-folded monomer in solution. (A) AFM imaging of hCdc45 reveals a homogeneous population of monomeric protein molecules in solution. The scale bar corresponds to 100 nm. The enlarged inset shows examples of elongated Cdc45 shapes. (B) AFM volume analysis indicates a molecular mass of 63 kDa for human Cdc45, consistent with a homogeneous population of monomeric protein molecules in solution. AFM volumes are translated into protein molecular weight based on a linear calibration curve (inset).
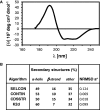
Synchrotron radiation circular dichroism spectrum of hCdc45. (A) The spectrum was recorded at the UV-CD12 beamline of the ANKA storage ring, Karlsruhe, Germany, in 10 mM potassium phosphate, pH 7.5, and 150 mM NaF. The ellipticities θ, in deg dmol-cm, is plotted as a function of wavelength. Data processing was carried out using the CDtool software. (B) The secondary structure elements of hCdc45 were assessed using the indicated algorithms and the SP175 reference dataset, available on the DichroWeb server. An asterisk indicates the normalized root-mean-square deviation between calculated and experimental spectra.
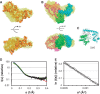
Three-dimensional structural models of hCdc45 protein. (A) Superposition of the GASBOR and DAMMIF models. (B) Superposition of the DAMMIF and BUNCH models. The ab initio bead model of hCdc45 in (A) and (B) is presented as transparent yellow spheres, the model from GASBOR as an orange surface, the model from BUNCH as a molecular surface with the N-terminal domain in green, the C-terminal domain in turquoise and the helical insertion or loop in magenta. The two figures are related by a rotation of 90° around the abscissa. (C) The crystal structure of T. thermophilus RecJ (1IR6) with the same coloring as in the BUNCH model for comparison. The BUNCH model is based on the N-and C-terminal domains of RecJ, whereas the gray part, corresponding to the linker and insertion, was rebuilt in the modeling. (D) SAXS curve of hCdc45. The experimental SAXS profile of human Cdc45 protein (black dots) is compared with the theoretical scattering curves calculated from the BUNCH model (red), the DAMMIF model (blue) and the RecJ crystal structure (1IR6) (green). (E) Guinier plot of hCdc45. The linearity of the Guiner plot (plotted in the range of 0.8 < sRg < 1.3) indicates the quality of the experimental SAXS data.
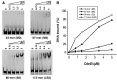
Binding of hCdc45 to ssDNA substrates. (A) Electrophoretic mobility shift assays were performed with 2 nM 5′-labeled ssDNA and the indicated amounts of hCdc45. After 10 min at 30°C, the reaction mixtures were cooled to 0°C. After addition of 5-µl loading dye, the samples were electrophoresed through a 10% non-denaturing polyacrylamide gels in 1× TBE buffer. Subsequently, gels were dried and exposed to a phosphorimaging screen. Gels were visualized using a phosphor-imager (Typhoon Trio; GE Healthcare). (B) The graph represents the percentage of bound DNA per added concentration of hCdc45 as calculated by the Image Quant software.
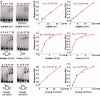
Binding of hCdc45 to different DNA structures. (A) Electrophoretic mobility shift assays were performed with 4 nM 5′-labeled DNA substrates and the indicated amounts of hCdc45. Gel electrophoresis was as described in the legend for Figure 4. The used oligonucleotides are listed in parentheses. The asterisks (*) represent the 5′-label. (B) Real-time binding analysis of various DNA substrates to hCdc45. Direct interaction between Cdc45 and DNA substrates was monitored using the BIAcore system. Binding was measured at 25°C at a flow rate of 30 µl/min in 20 mM HEPES-KOH, pH 7.5, 150 mM KCl, 1 mM DTT, 3 mM EDTA and the surfactant P20. The dissociation constants were calculated from the concentration-dependent steady-state response of DNA binding.
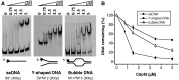
hCdc45 binding to branched DNA structures. (A) Electrophoretic mobility shift assays were performed using 2 nM 5′-labeled DNA substrates and the indicated amounts of hCdc45 as described in the legend for Figure 4. (B) The graph represents the percentage of unbound DNA as function of the hCdc45 concentration. The mean standard error from three independent experiments is also given. Densitometric analyses were performed using the Image-Quant software.
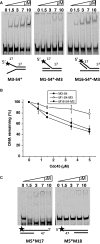
hCdc45 binds tightly to the 3′ arm of branched DNA structures. (A) hCdc45 binding to Y-shaped DNA and FLAP-DNA. Electrophoretic mobility shift assays were performed with 2-nM 5′-labeled DNA as described in the legend for Figure 4. (B) The graph represents the percentage of unbound DNA as function of the hCdc45 concentration with mean standard errors as described in the legend for Figure 6. (C) Binding of hCdc45 to 3′ and 5′-overhangs.
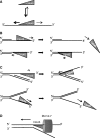
Hypothetical models for the action of hCdc45 on branched DNAs. (A) Binding of hCdc45 to ssDNA and sliding to the 5′ or 3′-end. (B) Preferred movement along ssDNA in the 3′–5′ direction aids to find the ss/ds junction (C) hCdc45 possesses a higher affinity for 5′-blocked flaps as compared with 3′-blocked structures. (D) Cdc45 may migrate in front of the Mcm2-7 helicase from 3′ to 5′ and, acting as a molecular ‘wedge’, to help displace the lagging strand.
Similar articles
-
Petojevic T, Pesavento JJ, Costa A, Liang J, Wang Z, Berger JM, Botchan MR. Petojevic T, et al. Proc Natl Acad Sci U S A. 2015 Jan 20;112(3):E249-58. doi: 10.1073/pnas.1422003112. Epub 2015 Jan 5. Proc Natl Acad Sci U S A. 2015. PMID: 25561522 Free PMC article.
-
Dpb11 protein helps control assembly of the Cdc45·Mcm2-7·GINS replication fork helicase.
Dhingra N, Bruck I, Smith S, Ning B, Kaplan DL. Dhingra N, et al. J Biol Chem. 2015 Mar 20;290(12):7586-601. doi: 10.1074/jbc.M115.640383. Epub 2015 Feb 6. J Biol Chem. 2015. PMID: 25659432 Free PMC article.
-
Krastanova I, Sannino V, Amenitsch H, Gileadi O, Pisani FM, Onesti S. Krastanova I, et al. J Biol Chem. 2012 Feb 3;287(6):4121-8. doi: 10.1074/jbc.M111.285395. Epub 2011 Dec 6. J Biol Chem. 2012. PMID: 22147708 Free PMC article.
-
The eukaryotic Mcm2-7 replicative helicase.
Vijayraghavan S, Schwacha A. Vijayraghavan S, et al. Subcell Biochem. 2012;62:113-34. doi: 10.1007/978-94-007-4572-8_7. Subcell Biochem. 2012. PMID: 22918583 Review.
-
Li H, O'Donnell ME. Li H, et al. Bioessays. 2018 Mar;40(3):10.1002/bies.201700208. doi: 10.1002/bies.201700208. Epub 2018 Feb 6. Bioessays. 2018. PMID: 29405332 Free PMC article. Review.
Cited by
-
Mechanisms and regulation of DNA replication initiation in eukaryotes.
Parker MW, Botchan MR, Berger JM. Parker MW, et al. Crit Rev Biochem Mol Biol. 2017 Apr;52(2):107-144. doi: 10.1080/10409238.2016.1274717. Epub 2017 Jan 17. Crit Rev Biochem Mol Biol. 2017. PMID: 28094588 Free PMC article. Review.
-
Testis transcriptome profiling identified lncRNAs involved in spermatogenic arrest of cattleyak.
Cai X, Wu S, Mipam T, Luo H, Yi C, Xu C, Zhao W, Wang H, Zhong J. Cai X, et al. Funct Integr Genomics. 2021 Nov;21(5-6):665-678. doi: 10.1007/s10142-021-00806-8. Epub 2021 Oct 9. Funct Integr Genomics. 2021. PMID: 34626308
-
Yi GS, Song Y, Wang WW, Chen JN, Deng W, Cao W, Wang FP, Xiao X, Liu XP. Yi GS, et al. Genes (Basel). 2017 Aug 24;8(9):211. doi: 10.3390/genes8090211. Genes (Basel). 2017. PMID: 28837073 Free PMC article.
-
Petojevic T, Pesavento JJ, Costa A, Liang J, Wang Z, Berger JM, Botchan MR. Petojevic T, et al. Proc Natl Acad Sci U S A. 2015 Jan 20;112(3):E249-58. doi: 10.1073/pnas.1422003112. Epub 2015 Jan 5. Proc Natl Acad Sci U S A. 2015. PMID: 25561522 Free PMC article.
-
Gekko K. Gekko K. Biophys Physicobiol. 2019 Feb 6;16:41-58. doi: 10.2142/biophysico.16.0_41. eCollection 2019. Biophys Physicobiol. 2019. PMID: 30923662 Free PMC article. Review.
References
-
- Araki H. Cyclin-dependent kinase-dependent initiation of chromosomal DNA replication. Curr. Opin. Cell. Biol. 2010;22:766–771. - PubMed
-
- MacNeill SA. Structure and function of the GINS complex, a key component of the eukaryotic replisome. Biochem. J. 2010;425:489–500. - PubMed
-
- Pospiech H, Grosse F, Pisani FM. The initiation step of eukaryotic DNA replication. Subcell Biochem. 2010;50:79–104. - PubMed
Publication types
MeSH terms
Substances
LinkOut - more resources
Full Text Sources
Other Literature Sources
Research Materials
Miscellaneous