DNA bridging and looping by HMO1 provides a mechanism for stabilizing nucleosome-free chromatin - PubMed
. 2014 Aug;42(14):8996-9004.
doi: 10.1093/nar/gku635. Epub 2014 Jul 24.
Affiliations
- PMID: 25063301
- PMCID: PMC4132745
- DOI: 10.1093/nar/gku635
DNA bridging and looping by HMO1 provides a mechanism for stabilizing nucleosome-free chromatin
Divakaran Murugesapillai et al. Nucleic Acids Res. 2014 Aug.
Abstract
The regulation of chromatin structure in eukaryotic cells involves abundant architectural factors such as high mobility group B (HMGB) proteins. It is not understood how these factors control the interplay between genome accessibility and compaction. In vivo, HMO1 binds the promoter and coding regions of most ribosomal RNA genes, facilitating transcription and possibly stabilizing chromatin in the absence of histones. To understand how HMO1 performs these functions, we combine single molecule stretching and atomic force microscopy (AFM). By stretching HMO1-bound DNA, we demonstrate a hierarchical organization of interactions, in which HMO1 initially compacts DNA on a timescale of seconds, followed by bridge formation and stabilization of DNA loops on a timescale of minutes. AFM experiments demonstrate DNA bridging between strands as well as looping by HMO1. Our results support a model in which HMO1 maintains the stability of nucleosome-free chromatin regions by forming complex and dynamic DNA structures mediated by protein-protein interactions.
© The Author(s) 2014. Published by Oxford University Press on behalf of Nucleic Acids Research.
Figures
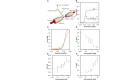
Characterization of the equilibrium binding constant and the cooperativity parameter using optical tweezers. (A) Schematic of optical tweezers. Phage-λ DNA (not to scale) is captured between two beads held on one side by two focused laser beams and on the other side by a micropipette. The DNA is stretched by moving the micropipette and measuring the resulting force with the optical tweezers. (B) The force-extension curve is obtained by stretching the DNA in the absence (black line) and in the presence of 1 nM HMO1 (orange line). The change in overstretching force in the presence of HMO1 proteins is indicated by ΔFov (C) To study the change in mechanical properties of the DNA in the presence of HMO1, data points (open symbols) in the presence (orange) and absence (black) of HMO1 are fit using the extensible WLC model (Equation 1, solid lines). (D) The persistence length versus concentration (symbols) is fit (dotted line) to Equation (4). (E) The contour length versus concentration (symbols) is fit (dotted line) to Equation (5). (F) The overstretching force versus concentration (symbols) is fit (dotted line) to Equation (6). The error bars indicate standard error of measurement for N ≥4 for all panels.
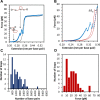
Breaking of loops formed by HMO1 proteins bound to phage-λ DNA characterized by optical tweezers. (A) Extension of phage-λ DNA curves are shown for phage-λ DNA in the absence (black) and presence (blue) of 0.3 nM HMO1 proteins when DNA was initially held at low forces (F < 1 pN). The extension curves show breaking of HMO1-bound DNA loops (observed jumps). (B) Extension curves of phage-λ DNA in the presence of 0.3 nM HMO1. Each jump represents an unbinding event. The loop size can be quantified by measuring the contour length change over the force jump by fitting to the WLC model (solid red lines). ΔBds represents the fitted length change, which is the loop size. We kept constant the value of persistence length and elastic modulus for these fits. (C) Histogram of loop sizes in base pairs. The most probable loop size corresponds to a range between 400 and 600 bp. (D) Histogram of loop breaking forces. The most probable force to break a loop is between 10 and 15 pN.
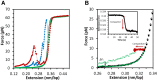
Dynamics of loop formation and compaction in the presence of HMO1. (A) Consecutive extension curves in the absence (black) and presence of HMO1 proteins (blue, green and red). The first extension curve (blue) shows DNA loop disruption, but the second extension curve (green) immediately after shows no DNA loops. However, after waiting for ∼5–7 min at F < 1 pN and then stretching a third time, loops have reformed at different locations, as shown by the red extension curve. (B) Compaction of a single DNA molecule by HMO1 determined by constant force measurement. The filled and open black circles show the extension and release curves in the absence of HMO1. The green filled circles show the extension of the DNA molecule before flowing the protein. This curve follows the black circles up to 10 pN, where the DNA is held and exposed to 10 nM HMO1. The red dots show the decrease in length at constant force as the DNA is compacted. The open green circles represent the release curve after the single DNA molecule has been held at constant force in the presence of HMO1 for several minutes. This curve does not overlap with the release curve in the absence of protein, showing that the DNA molecule has been compacted. The compaction force, or additional force due to protein binding in the limit of low extension, is measured to be ΔFc = 1.7 ± 0.3 pN (averaged over five DNA molecules). The inset shows the change in extension as a function of time during compaction (black). The rate of compaction is determined by fitting to a single exponential (red line) with k = 0.64 ± 0.10 s−1 (τ = 1.6 ± 0.2 s).
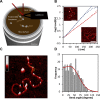
AFM images of linearized pBR322 DNA and DNA–HMO1 complexes deposited on a Mg2+-treated mica surface, revealing the global and local flexibility of the DNA in presence of HMO1. (A) Schematic of the AFM. The deflection of a laser beam is converted into a topographical imaging signal. Thus, HMO1–DNA complexes (not to scale) can be probed locally at the single molecule level. (B) Fits to 2D WLC model (Equation 7) allow us to determine the persistence length of DNA in the absence (red) and in the presence of HMO1 (blue). AFM images of 4361 base pair linearized plasmid DNA pBR322 in the absence of protein (lower right, scale bar 300 nm) and in the presence of protein (upper left image, white dots representing protein bound, scale bar 200 nm) are also shown. The concentration of HMO1 used is 3 nM and the concentration of DNA is 0.11 nM. (C) A three-dimensional representation of locally probed HMO1–DNA complexes. AFM images make it possible to resolve protein-bound sites from DNA only. Binding of the proteins is observed both at DNA termini and at sites of protein-induced DNA bends. The color bar represents the sample height ranging from 0.0 to 2.0 nm. (D) The distribution of HMO1-induced DNA bend angles is shown, along with its bi-Gaussian fit (red curve).
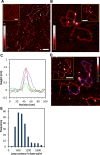
Architectural role of HMO1 in bridging, looping and compacting DNA. (A) Two-dimensional representation of bridges and loops mediated by HMO1 (scale bar 200 nm). We imaged 174 DNA molecules, 70% of the molecules had bridges and, on average, there were two bridges per molecule. The concentration of HMO1 used is 3 nM and the concentration of DNA is 0.11 nM. The inset shows the linearized plasmid DNA pBR322 in the absence of protein (scale bar 300 nm). (B) Three-dimensional representation of a looped single DNA molecule. Cross sections of DNA only, DNA with proteins bound, proteins bridging two strands of DNA and bridged DNA are shown in green, red, purple and blue, respectively. The inset represents a two-dimensional representation of locally probed HMO1–DNA complexes (scale bar 100 nm). (C) Graphs of the heights are shown for each cross section on the image in (B). (D) A two-dimensional representation of a single DNA molecule displaying multiple loops and bridges mediated by HMO1 with loops resulting from computation tracing shown in blue. Inset: Original AFM image without traces (scale bar 100 nm). (E) Histogram of loop sizes mediated by HMO1. The color bar in each panel represents the sample height ranging from 0.0 to 2.0 nm.
Similar articles
-
Yeast HMO1: Linker Histone Reinvented.
Panday A, Grove A. Panday A, et al. Microbiol Mol Biol Rev. 2016 Nov 30;81(1):e00037-16. doi: 10.1128/MMBR.00037-16. Print 2017 Mar. Microbiol Mol Biol Rev. 2016. PMID: 27903656 Free PMC article. Review.
-
Single and double box HMGB proteins differentially destabilize nucleosomes.
McCauley MJ, Huo R, Becker N, Holte MN, Muthurajan UM, Rouzina I, Luger K, Maher LJ 3rd, Israeloff NE, Williams MC. McCauley MJ, et al. Nucleic Acids Res. 2019 Jan 25;47(2):666-678. doi: 10.1093/nar/gky1119. Nucleic Acids Res. 2019. PMID: 30445475 Free PMC article.
-
[HMGB Proteins as DNA Chaperones That Modulate Chromatin Activity].
Kozlova AL, Valieva ME, Maluchenko NV, Studitsky VM. Kozlova AL, et al. Mol Biol (Mosk). 2018 Sep-Oct;52(5):737-749. doi: 10.1134/S0026898418050099. Mol Biol (Mosk). 2018. PMID: 30363049 Review. Russian.
-
Kamau E, Bauerle KT, Grove A. Kamau E, et al. J Biol Chem. 2004 Dec 31;279(53):55234-40. doi: 10.1074/jbc.M409459200. Epub 2004 Oct 25. J Biol Chem. 2004. PMID: 15507436
-
Hmo1: A versatile member of the high mobility group box family of chromosomal architecture proteins.
Bi X. Bi X. World J Biol Chem. 2024 Aug 12;15(1):97938. doi: 10.4331/wjbc.v15.i1.97938. World J Biol Chem. 2024. PMID: 39156122 Free PMC article. Review.
Cited by
-
Panday A, Xiao L, Grove A. Panday A, et al. Nucleic Acids Res. 2015 Jul 13;43(12):5759-70. doi: 10.1093/nar/gkv498. Epub 2015 May 15. Nucleic Acids Res. 2015. PMID: 25979266 Free PMC article.
-
Atomic Force Microscopy Characterization of Reconstituted Protein-DNA Complexes.
Cajili MKM, Prieto EI. Cajili MKM, et al. Methods Mol Biol. 2024;2819:279-295. doi: 10.1007/978-1-0716-3930-6_14. Methods Mol Biol. 2024. PMID: 39028512
-
Establishment and Maintenance of Open Ribosomal RNA Gene Chromatin States in Eukaryotes.
Schächner C, Merkl PE, Pilsl M, Schwank K, Hergert K, Kruse S, Milkereit P, Tschochner H, Griesenbeck J. Schächner C, et al. Methods Mol Biol. 2022;2533:25-38. doi: 10.1007/978-1-0716-2501-9_2. Methods Mol Biol. 2022. PMID: 35796980 Free PMC article. Review.
-
Franco-Losilla M, Nordzieke S, Feldmann I, Limón MC, Avalos J. Franco-Losilla M, et al. Genes (Basel). 2023 Aug 21;14(8):1661. doi: 10.3390/genes14081661. Genes (Basel). 2023. PMID: 37628712 Free PMC article.
-
Kasahara K, Nakayama R, Shiwa Y, Kanesaki Y, Ishige T, Yoshikawa H, Kokubo T. Kasahara K, et al. PLoS Genet. 2020 Jun 30;16(6):e1008865. doi: 10.1371/journal.pgen.1008865. eCollection 2020 Jun. PLoS Genet. 2020. PMID: 32603360 Free PMC article.
References
-
- Štros M. HMGB proteins: interactions with DNA and chromatin. Biochim. Biophys. Acta. 2010;1799:101–113. - PubMed
-
- Bianchi M.E. HMGB1 loves company. J. Leukoc. Biol. 2009;86:573–576. - PubMed
-
- Thomas J.O., Travers A.A. HMG1 and 2, and related ‘architectural’ DNA-binding proteins. Trends Biochem. Sci. 2001;26:167–174. - PubMed
Publication types
MeSH terms
Substances
Grants and funding
LinkOut - more resources
Full Text Sources
Other Literature Sources
Molecular Biology Databases
Miscellaneous