Quantitative profiling of initiating ribosomes in vivo - PubMed
Quantitative profiling of initiating ribosomes in vivo
Xiangwei Gao et al. Nat Methods. 2015 Feb.
Abstract
Cells have evolved exquisite mechanisms to fine-tune the rate of protein synthesis in response to stress. Systemic mapping of start-codon positions and precise measurement of the corresponding initiation rate would transform our understanding of translational control. Here we present quantitative translation initiation sequencing (QTI-seq), with which the initiating ribosomes can be profiled in real time at single-nucleotide resolution. Resultant initiation maps not only delineated variations of start-codon selection but also highlighted a dynamic range of initiation rates in response to nutrient starvation. The integrated data set provided unique insights into principles of alternative translation and mechanisms controlling different aspects of translation initiation. With RiboTag mice, QTI-seq permitted tissue-specific profiling of initiating ribosomes in vivo. Liver cell-specific ribosome profiling uncovered a robust translational reprogramming of the proteasome system in fasted mice. Our findings illuminated the prevalence and dynamic nature of translational regulation pivotal to physiological adaptation in vivo.
Conflict of interest statement
Competing Financial Interests: The authors declare no competing financial interests.
Figures
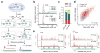
(a) Schematic of Ribo-seq (left panel) and QTI-seq (right panel) procedures. The red line represents the ribosome density on average based on regular Ribo-seq, whereas the blue line indicates the density of initiating ribosome obtained from QTI-seq. (b) Meta-gene analysis of CHX-associated ribosome density (top panel) and LTM-associated ribosome density (bottom panel) in HEK293 cells captured by QTI-seq. Normalized RPF reads are averaged across the entire transcriptome and aligned at the annotated start codon. Different reading frames are separated and color coded. (c) A stacked bar plot showing the relative ratio of different types of TIS identified by GTI-seq or QTI-seq in HEK293 cells. (d) A scatter plot showing the correlation between LTM-associated aTIS density normalized by upper quartile and CHX-associated CDS ribosome occupancy normalized by RPKM. Genes with single annotated TIS or multiple TISs are shown in blue and red dots respectively. (e) Examples of single TIS (ABCD3) and multiple TIS (GEMIN6) genes revealed by GTI-seq (top panel) and QTI-seq (bottom panel). The same scale is used for Y-axis. The corresponding gene structure is shown below the X-axis.
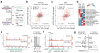
(a) Schematic of experimental procedures for RNA-seq, Ribo-seq, and QTI-seq in cells with or without starvation. (b) A scatter plot of fold changes in LTM-associated aTIS density and CHX-associated CDS ribosome occupancy in HEK293 cells before and after amino acid starvation. Genes with single annotated TIS or multiple TISs are shown in blue and red dots respectively. Genes with more than 1.5 fold changes are marked with “Increase” or “Decrease” boxes. (c) A scatter plot of fold changes in LTM-associated aTIS density and CHX-associated CDS ribosome occupancy in MEF cells before and after amino acid starvation. (d) A heatmap of false discovery rate (FDR) of enriched GO terms (biological process) for gene groups with translational downregulation (Dec) or upregulation (Inc) in response to amino acid starvation. (e) An example of genes (RPS28) with translational downregulation after amino acid starvation. The same scale is used for Y-axis. The corresponding gene structure is shown below the X-axis. The right panel is a bar graph depicting the relative RNA abundance, CDS ribosome occupancy, and aTIS ribosome density. (f) An example of multi-TIS genes (GADD45G) showing an increased aTIS fraction after amino acid starvation. (g) Experimental validation of translational control of GADD45G by a Fluc reporter bearing the 5′UTR of GADD45G with (WT) or without the uTIS codon CUG (Del). (means ± SEM; n = 3; * p < 0.05 student t-test).
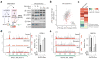
(a) Schematic of mTORC1 signaling and eIF2α phosphorylation in controlling translation initiation. Right panel shows immunoblotting results of eIF2α(S51D) cells with either amino acid starvation or Dox-induced eIF2α(S51D) expression. (b) A scatter plot of fold changes in LTM-associated aTIS density in eIF2α(S51D) cells between amino acid starvation and Dox-induced eIF2α(S51D) expression. TOP mRNAs are shown in Red dots. (c) A heatmap of fold change of enriched GO terms (biological process) for gene groups with translational downregulation (green) or upregulation (red) in response to amino acid starvation (left) or Dox-induced eIF2α(S51D) expression (right). (d) An example of genes (RPS11) showing translational repression in response to amino acid starvation but not Dox-induced eIF2α(S51D) expression. The right panel is validation of RPS11 translational control by a Fluc reporter bearing the 5′UTR of RPS11. (means ± SEM; n = 3; * p < 0.01 student t-test). (e) An example of genes (NUP43) showing translational upregulation in response to either amino acid starvation or Dox-induced eIF2α(S51D) expression. The right panel is validation of NUP43 translational control by a Fluc reporter bearing the 5′UTR of NUP43. (means ± SEM; n = 3; * p < 0.01 student t-test).
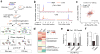
(a) Schematic of tissue-specific QTI-seq procedures using liver-specific RiboTag mice. (b) Meta-gene analysis of LTM-associated ribosome density (top panel) or CHX-associated ribosome density (bottom panel) in MEF cells (blue line) and liver cells (red line). Normalized RPF reads are averaged across the entire transcriptome and aligned at the annotated start codons and stop codons. (c) A scatter plot of fold changes in LTM-associated aTIS density and CHX-associated CDS ribosome occupancy in mouse liver cells with and without fasting. (d) A heatmap of fold changes for gene groups with translational downregulation (green) or upregulation (red) in fasted liver (left) or starved MEF cells (right). (e) Reporter assay using an in vitro translation system reprogrammed from mouse liver lysates with or without fasting. The relative translation efficiency of a synthesized Fluc mRNA containing 5′UTRs of PSMA3 or PSMB4 is shown in bar graph (means ± SEM; n = 3; * p < 0.01 student t-test). (f) Mice of 8-12 week old were treated with or without overnight fasting. The chymotrypsin activity of liver homogenates was measured by Proteasome-Glo (means ± SEM; n = 3; * p < 0.01 student t-test).
Similar articles
-
Genome-Wide Profiling of Alternative Translation Initiation Sites.
Gao X, Wan J, Qian SB. Gao X, et al. Methods Mol Biol. 2016;1358:303-16. doi: 10.1007/978-1-4939-3067-8_19. Methods Mol Biol. 2016. PMID: 26463392
-
N6-Methyladenosine Guides mRNA Alternative Translation during Integrated Stress Response.
Zhou J, Wan J, Shu XE, Mao Y, Liu XM, Yuan X, Zhang X, Hess ME, Brüning JC, Qian SB. Zhou J, et al. Mol Cell. 2018 Feb 15;69(4):636-647.e7. doi: 10.1016/j.molcel.2018.01.019. Epub 2018 Feb 8. Mol Cell. 2018. PMID: 29429926 Free PMC article.
-
Global mapping of translation initiation sites in mammalian cells at single-nucleotide resolution.
Lee S, Liu B, Lee S, Huang SX, Shen B, Qian SB. Lee S, et al. Proc Natl Acad Sci U S A. 2012 Sep 11;109(37):E2424-32. doi: 10.1073/pnas.1207846109. Epub 2012 Aug 27. Proc Natl Acad Sci U S A. 2012. PMID: 22927429 Free PMC article.
-
Insights into the mechanisms of eukaryotic translation gained with ribosome profiling.
Andreev DE, O'Connor PB, Loughran G, Dmitriev SE, Baranov PV, Shatsky IN. Andreev DE, et al. Nucleic Acids Res. 2017 Jan 25;45(2):513-526. doi: 10.1093/nar/gkw1190. Epub 2016 Dec 6. Nucleic Acids Res. 2017. PMID: 27923997 Free PMC article. Review.
-
Engineering bacterial translation initiation - Do we have all the tools we need?
Vigar JRJ, Wieden HJ. Vigar JRJ, et al. Biochim Biophys Acta Gen Subj. 2017 Nov;1861(11 Pt B):3060-3069. doi: 10.1016/j.bbagen.2017.03.008. Epub 2017 Mar 14. Biochim Biophys Acta Gen Subj. 2017. PMID: 28315412 Review.
Cited by
-
Mechanisms of protein balance in skeletal muscle.
Anthony TG. Anthony TG. Domest Anim Endocrinol. 2016 Jul;56 Suppl(Suppl):S23-32. doi: 10.1016/j.domaniend.2016.02.012. Domest Anim Endocrinol. 2016. PMID: 27345321 Free PMC article. Review.
-
riboWaltz: Optimization of ribosome P-site positioning in ribosome profiling data.
Lauria F, Tebaldi T, Bernabò P, Groen EJN, Gillingwater TH, Viero G. Lauria F, et al. PLoS Comput Biol. 2018 Aug 13;14(8):e1006169. doi: 10.1371/journal.pcbi.1006169. eCollection 2018 Aug. PLoS Comput Biol. 2018. PMID: 30102689 Free PMC article.
-
Ribosome Profiling: Global Views of Translation.
Ingolia NT, Hussmann JA, Weissman JS. Ingolia NT, et al. Cold Spring Harb Perspect Biol. 2019 May 1;11(5):a032698. doi: 10.1101/cshperspect.a032698. Cold Spring Harb Perspect Biol. 2019. PMID: 30037969 Free PMC article. Review.
-
Human La binds mRNAs through contacts to the poly(A) tail.
Vinayak J, Marrella SA, Hussain RH, Rozenfeld L, Solomon K, Bayfield MA. Vinayak J, et al. Nucleic Acids Res. 2018 May 4;46(8):4228-4240. doi: 10.1093/nar/gky090. Nucleic Acids Res. 2018. PMID: 29447394 Free PMC article.
-
Bayesian prediction of RNA translation from ribosome profiling.
Malone B, Atanassov I, Aeschimann F, Li X, Großhans H, Dieterich C. Malone B, et al. Nucleic Acids Res. 2017 Apr 7;45(6):2960-2972. doi: 10.1093/nar/gkw1350. Nucleic Acids Res. 2017. PMID: 28126919 Free PMC article.
References
-
- Aitken CE, Lorsch JR. A mechanistic overview of translation initiation in eukaryotes. Nat Struct Mol Biol. 2012;19:568–576. - PubMed
-
- Gray NK, Wickens M. Control of translation initiation in animals. Annu Rev Cell Dev Biol. 1998;14:399–458. - PubMed
-
- Spriggs KA, Bushell M, Willis AE. Translational regulation of gene expression during conditions of cell stress. Mol Cell. 2010;40:228–237. - PubMed
Publication types
MeSH terms
Substances
Grants and funding
LinkOut - more resources
Full Text Sources
Other Literature Sources