The DNA-dependent protein kinase: A multifunctional protein kinase with roles in DNA double strand break repair and mitosis - PubMed
Review
The DNA-dependent protein kinase: A multifunctional protein kinase with roles in DNA double strand break repair and mitosis
Nicholas Jette et al. Prog Biophys Mol Biol. 2015 Mar.
Abstract
The DNA-dependent protein kinase (DNA-PK) is a serine/threonine protein kinase composed of a large catalytic subunit (DNA-PKcs) and the Ku70/80 heterodimer. Over the past two decades, significant progress has been made in elucidating the role of DNA-PK in non-homologous end joining (NHEJ), the major pathway for repair of ionizing radiation-induced DNA double strand breaks in human cells and recently, additional roles for DNA-PK have been reported. In this review, we will describe the biochemistry, structure and function of DNA-PK, its roles in DNA double strand break repair and its newly described roles in mitosis and other cellular processes.
Keywords: DNA-dependent protein kinase; Mitosis; Non-homologous end joining.
Copyright © 2014 Elsevier Ltd. All rights reserved.
Conflict of interest statement
The authors declare they have no conflict of interest.
Figures
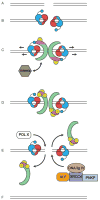
A. DSBs can be formed either by DNA damaging agents such as ionizing radiation (IR) or during the process of V(D)J recombination in the vertebrate immune system. B. DSBs are detected by the Ku70/80 heterodimer, which binds to the ends of the DSB. Ku70 (shown in red) is positioned proximal to the DSB end and Ku80 (shown in blue) is distal to the end (Walker et al., 2001). The C-terminal domain of Ku80 is composed of a flexible linker with a globular domain and a C-terminal region that interacts directly with DNA-PKcs (Gell and Jackson, 1999), (shown as small blue circle). Significantly, SAXS revealed that this flexible domain extends towards Ku70, positioning the DNA-PKcs interacting region close to the end of the DSB (Hammel et al., 2010b). Ku plays a key role in NHEJ, being responsible for recruitment of multiple downstream proteins, including DNA-PKcs and XLF, to the DSB (reviewed in (Radhakrishnan et al., 2014; Wang and Lees-Miller, 2013)). C. Upon activation, DNA-PKcs is recruited to the DSB end, causing Ku to move inwards (Frit et al., 2000) (indicated by arrows) such that DNA-PKcs is now positioned at the extreme end of the DSB (Yoo and Dynan, 1999). dsDNA interacts with the central region of DNA-PKcs (Boskovic et al., 2003; Williams et al., 2008). It has been suggested that DNA-PKcs may open or fray the ends of the dsDNA which may allow single stranded DNA to bind in a channel in the head domain of DNA-PKcs (DeFazio et al., 2002) (see also Figure 2B). DNA-PKcs also interacts with Artemis (shown in gray), an endonuclease that, in the presence of DNA-PKcs, facilitates DNA hairpin opening at coding joints in V(D)J recombination (Goodarzi et al., 2006). The role of Artemis in repair of IR-induced DSBs is not known. DNA-PKcs is coloured as in Figure 2A, where the N-terminal HEAT repeats are in green, the FAT and FATC domains in magenta and the kinase domain in yellow, as described by (Sibanda et al., 2010). D. DNA-PKcs undergoes extensive autophosphorylation (yellow circles with P), which in vitro has been shown to induce large conformational changes and promote release of phosphorylated DNA-PKcs from the Ku-DNA complex (Chan and Lees-Miller, 1996; Dobbs et al., 2010; Hammel et al., 2010b). A recent study suggests that recruitment of the XRCC4-DNA ligase IV complex to the DSB is required for phosphorylation of DNA-PKcs (Cottarel et al., 2013). Artemis has also been reported to interact with DNA ligase IV (Malu et al., 2012; Ochi et al., 2013) (not shown in figure). E. DNA-end processing enzymes such as polynucleotide kinase/phosphatase (PNKP), which interacts with XRCC4 (Koch et al., 2004) and DNA polymerases mu and/or lambda of the pol X family are also recruited to the DSB, as is the XRCC4-DNA ligase IV-XLF complex, which seals the DSB ends. XRCC4 and XLF form long helical filaments (not shown in figure) that may facilitate bridging of ends prior to ligation (Hammel et al., 2011; Hammel et al., 2010a; Mahaney et al., 2013). F. NHEJ is completed when the processed ends of the DSB are covalently joined. How and when Ku is removed from DSB ends is not known precisely but may involve ubiquitination (Feng and Chen, 2012; Postow, 2011; Postow et al., 2008).
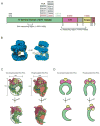
A. Cartoon of architecture of DNA-PKcs showing the N terminal HEAT repeat region (green), and the FAT and FAT-C domains (magenta) that flank the kinase domain (yellow). The major in vivo phosphorylation sites discussed in this review are indicated above the figure and the domain boundaries by the numbers below. See also (Dobbs et al., 2010). Mitotic phosphorylation sites (indicated in red) are from (Douglas et al., 2014) and (Lee et al., 2011). The region of DNA-PKcs implicated in interaction with Ku and DNA are from (Jin et al., 1997) and (Gupta and Meek, 2005), respectively. Also shown are the caspase cleavage sites (green) reported in (Song et al., 1996). B. Representation of the cryo-electron microscopy structure of DNA-PKcs from (Williams et al., 2008) showing the potential path of double stranded DNA through the large central cavity, and a model for the single stranded ends of frayed DNA through the putative upper central channel of the DNA-PKcs molecule. On the left, DNA-PKcs is shown with the crown (now known to contain the FAT-kinase and FATC domains) on the top, and the HEAT repeats on the sides, forming the arms that surround the large central cavity. In the representation shown, dsDNA is shown to the left in the central cavity. The structure on the right is rotated by 90°, illustrating the potential path of single stranded DNA into the head domain as suggested in (Williams et al., 2008). This figure is reproduced from (Williams et al., 2008) with permission. C. Upper two panels: Representations of the crystal structure of DNA-PKcs from (Sibanda et al., 2010) coloured as in panel A (HEAT repeats in green, FAT-kinase and FATC in magenta-yellow-magenta), superimposed on the SAXS envelopes of non-phosphorylated (cream) and autophosphorylated (brown) DNA-PKcs from (Hammel et al., 2010b) and (Dobbs et al., 2010). The lower two panels represent the structures rotated by 90°. D. Hypothetical models for DNA-PKcs in its non-phosphorylated and autophosphorylated forms. In the lower panel, the hypothetical structures are rotated by 90°. We suggest that autophosphorylation of DNA-PKcs may induce opening of the putative gap at the base of DNA-PKcs, as reported in (Sibanda et al., 2010) (top panels) or a conformational change, perhaps with opening or elongation of the concave surface created by the HEAT domain (lower panels). Colours are as in panel A. See text and (Dobbs et al., 2010; Hammel et al., 2010b) for details.
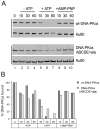
A. Human wild type-DNA-PKcs and DNA-PKcs in which serines and threonines at the ABCDE phosphorylation sites (T2609, S2612, T2620, S2624, T2638, T2647) were mutated to alanine, were stably expressed in DNA-PKcs-deficient V3 rodent cells, purified and incubated with biotin-labelled dsDNA either in the absence of ATP, in the presence of ATP, or the presence of the non-hydrolysable ATP analogue, AMP-PNP as described in (Hammel et al., 2010b). After washing with buffer, beads were resuspended in SDS sample buffer and analysed by SDS PAGE and immunoblot using antibodies to DNA-PKcs or Ku80 as shown. The results show that phosphorylation results in loss of wild-type DNA-PKcs from Ku-DNA complexes whereas more DNA-PKcs is retained with Ku-DNA complexes in the presence of ATP in the autophosphorylation defective mutant (ABCDE sites mutated to alanine). See text and (Hammel et al., 2010b) for details. The upper panel (interaction of wt-DNA-PKcs with Ku and DNA) is taken from Supplementary Figure 10 in (Hammel et al., 2010b), permission applied for. The experiment in the lower panel (purified DNA-PKcs with ABCDE phosphorylation sites mutated to alanine, ABCDE>A) was carried out at the same time as the experiment in the upper panel and equivalent exposures are shown. B. Bands corresponding to DNA-PKcs in panel A were quantitated using Quantity One software (Biorad), normalized to Ku80 and expressed as a percentage of the sample in lane 1 (incubated with no ATP).
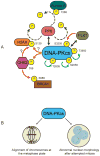
A. DNA-PKcs is autophosphorylated (green arrows) on multiple sites in mitosis (Douglas et al., 2014; Lee et al., 2011). DNA-PKcs also interacts with protein phosphatase 6 (PP6) (double headed black arrow) in asynchronously growing and nocodazole-treated mitotic cells (Douglas et al., 2010; Mi et al., 2009). PP6 dephosphorylates T288 of mitotic protein kinase Aurora A in mitosis (Zeng et al., 2010) and serine 139 of H2AX after IR (Douglas et al., 2010) (dashed lines), but whether these two events are related to its ability to interact with DNA-PKcs is unknown. DNA-PKcs interacts with polo-like kinase (PLK1) (double headed black arrow) and PLK1 phosphorylates DNA-PKcs on serine 3205 in mitosis (red arrow). S3205 is dephosphorylated by PP6 (dashed lines) in mitosis and after IR (Douglas et al., 2014). Also shown is DNA-PKcs-dependent phosphorylation of Chk2 on threonine 68 and histone H2AX on serine 139 in mitosis (red arrows) (see Figure 5). In vivo DNA-PKcs may phosphorylate histone H2AX either directly or indirectly through phosphorylation and activation of Chk2 (Tu et al., 2013). DNA-PK-dependent activation of Chk2 also leads to phosphorylation of BRCA1 on serine 988 in mitosis, which is required for accurate mitosis and genomic stability (Shang et al., 2014). B. Depletion or inhibition of DNA-PKcs results in misaligned mitotic chromosomes and abnormal nuclear morphologies suggesting that DNA-PKcs is required for correct alignment of mitotic chromosomes at the metaphase plate (left) and to prevent abnormal segregation of nuclear contents in cytokinesis.
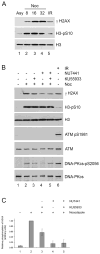
A. HeLa cells were either grown under asynchronous conditions (Asy, lane 1), or incubated with nocodazole (Noc, 40ng/ml) for 8, 16 or 32 hours as indicated (lanes 2–5). For lanes 2–5, mitotic cells were collected by mitotic shake off and allowed to recover in the absence of nocodazole for 35 minutes. Cells were lysed in NETN buffer and H2AX was extracted from the chromatin pellets with 1% SDS as described in (Douglas et al., 2014; Douglas et al., 2010). 50 μg of extract was run on SDS PAGE, transferred to membrane and probed with antibodies to serine 139-phosphorylated histone H2AX (γ-H2AX), serine 10 phosphorylated histone H3 (H3pS10) (a marker of mitosis) and total H3 as indicated. Lane 5 contains 50 μg of extract from asynchronously growing HeLa cells harvested 1 hour after exposure to 10 Gy IR as a control. B. Hela cells were either grown under asynchronous conditions (lane 1) or treated with nocodazole (40 ng/ml) (lanes 2–5). After 15 hours, either DMSO control (lane 2), the ATM inhibitor KU55933 at 5 μM (lane 3), the DNA-PK inhibitor NU7441 at 8 μM (lane 4), or both KU55933 and NU7441 (lane 5) was added to the media. After 1 hour, mitotic cells were harvested by mitotic shake off as described in (Douglas et al., 2014) and mitotic cells were incubated for a further 1 hour with DMSO, KU55933 and/or NU7441 as above but in the absence of nocodazole. Cells were lysed in NETN buffer, H2AX was extracted from the chromatin pellet of NETN lysates as described above and 50 μg of protein was analysed on SDS PAGE gels followed by immunoblot as described previously (Douglas et al., 2010). Lane 6 contained 50 μg of protein from NETN extracts generated from asynchronously growing cells that had been irradiated 10 Gy, and allowed to recover for 1 hour as a control. See (Douglas et al., 2014) for details. C. Serine 139-H2AX phosphorylation from panel B was quantitated as in Figure 3, and normalized to total histone H3 staining. Relative phosphorylation of histone γ-H2AX in lanes 1–5 was normalized to phosphorylation with nocodazole treatment alone (lane 2). Results show the average of three independent experiments with standard deviation. Statistical analysis was carried out using the Student’s T test. In the presence of the ATM inhibitor KU55933, H2AX phosphorylation decreased by 43.3 +/− 13.0% (p = 0.029), in the presence of the DNA-PK inhibitor it decreased by 85.1+/− 10.6% (p = 0.005), and in the presence of both inhibitors combined it decreased by 83.3 +/− 13.5% (p = 0.008). Absence of ATM serine 1981 phosphorylation and presence of nocodazole-induced phosphorylation of DNA-PKcs on serine 2056 confirm results reported in (Douglas et al., 2014).
Similar articles
-
Gustafsson AS, Abramenkovs A, Stenerlöw B. Gustafsson AS, et al. Mutat Res. 2014 Nov;769:1-10. doi: 10.1016/j.mrfmmm.2014.06.004. Epub 2014 Jun 22. Mutat Res. 2014. PMID: 25771720
-
A novel small molecule inhibitor of the DNA repair protein Ku70/80.
Weterings E, Gallegos AC, Dominick LN, Cooke LS, Bartels TN, Vagner J, Matsunaga TO, Mahadevan D. Weterings E, et al. DNA Repair (Amst). 2016 Jul;43:98-106. doi: 10.1016/j.dnarep.2016.03.014. Epub 2016 Apr 7. DNA Repair (Amst). 2016. PMID: 27130816
-
DNA-PK-dependent phosphorylation of Ku70/80 is not required for non-homologous end joining.
Douglas P, Gupta S, Morrice N, Meek K, Lees-Miller SP. Douglas P, et al. DNA Repair (Amst). 2005 Aug 15;4(9):1006-18. doi: 10.1016/j.dnarep.2005.05.003. DNA Repair (Amst). 2005. PMID: 15941674
-
Repair of ionizing radiation-induced DNA double-strand breaks by non-homologous end-joining.
Mahaney BL, Meek K, Lees-Miller SP. Mahaney BL, et al. Biochem J. 2009 Feb 1;417(3):639-50. doi: 10.1042/BJ20080413. Biochem J. 2009. PMID: 19133841 Free PMC article. Review.
-
Mechanisms of DNA double strand break repair and chromosome aberration formation.
Iliakis G, Wang H, Perrault AR, Boecker W, Rosidi B, Windhofer F, Wu W, Guan J, Terzoudi G, Pantelias G. Iliakis G, et al. Cytogenet Genome Res. 2004;104(1-4):14-20. doi: 10.1159/000077461. Cytogenet Genome Res. 2004. PMID: 15162010 Review.
Cited by
-
Dynamic structures in DNA damage responses & cancer.
Tainer JA. Tainer JA. Prog Biophys Mol Biol. 2015 Mar;117(2-3):129-133. doi: 10.1016/j.pbiomolbio.2015.04.003. Prog Biophys Mol Biol. 2015. PMID: 25934179 Free PMC article. No abstract available.
-
Zhou H, Zhu P, Wang J, Toan S, Ren J. Zhou H, et al. Signal Transduct Target Ther. 2019 Dec 6;4:56. doi: 10.1038/s41392-019-0094-1. eCollection 2019. Signal Transduct Target Ther. 2019. PMID: 31839999 Free PMC article.
-
Becker A, Krebs-Brown A, Vetter C, Reuter T, Rodriguez-Gutierrez A, You X, Lissy M. Becker A, et al. Clin Transl Sci. 2023 Dec;16(12):2628-2639. doi: 10.1111/cts.13657. Epub 2023 Oct 31. Clin Transl Sci. 2023. PMID: 37905356 Free PMC article. Clinical Trial.
-
Two-Stage Synapsis of DNA Ends during Non-homologous End Joining.
Graham TG, Walter JC, Loparo JJ. Graham TG, et al. Mol Cell. 2016 Mar 17;61(6):850-8. doi: 10.1016/j.molcel.2016.02.010. Mol Cell. 2016. PMID: 26990988 Free PMC article.
-
XLF acts as a flexible connector during non-homologous end joining.
Carney SM, Moreno AT, Piatt SC, Cisneros-Aguirre M, Lopezcolorado FW, Stark JM, Loparo JJ. Carney SM, et al. Elife. 2020 Dec 8;9:e61920. doi: 10.7554/eLife.61920. Elife. 2020. PMID: 33289484 Free PMC article.
References
-
- Ahn JY, Schwarz JK, Piwnica-Worms H, Canman CE. Threonine 68 phosphorylation by ataxia telangiectasia mutated is required for efficient activation of Chk2 in response to ionizing radiation. Cancer Res. 2000;60:5934–6. - PubMed
-
- Anderson CW. DNA damage and the DNA-activated protein kinase. Trends Biochem Sci. 1993;18:433–7. - PubMed
-
- Anderson CW, Lees-Miller SP. The nuclear serine/threonine protein kinase DNA-PK. Crit Rev Eukaryot Gene Expr. 1992;2:283–314. - PubMed
-
- Bailey SM, Cornforth MN, Ullrich RL, Goodwin EH. Dysfunctional mammalian telomeres join with DNA double-strand breaks. DNA Repair (Amst) 2004;3:349–57. - PubMed
Publication types
MeSH terms
Substances
LinkOut - more resources
Full Text Sources
Other Literature Sources
Miscellaneous