Mapping Nucleosome Resolution Chromosome Folding in Yeast by Micro-C - PubMed
- ️Thu Jan 01 2015
Mapping Nucleosome Resolution Chromosome Folding in Yeast by Micro-C
Tsung-Han S Hsieh et al. Cell. 2015.
Abstract
We describe a Hi-C-based method, Micro-C, in which micrococcal nuclease is used instead of restriction enzymes to fragment chromatin, enabling nucleosome resolution chromosome folding maps. Analysis of Micro-C maps for budding yeast reveals abundant self-associating domains similar to those reported in other species, but not previously observed in yeast. These structures, far shorter than topologically associating domains in mammals, typically encompass one to five genes in yeast. Strong boundaries between self-associating domains occur at promoters of highly transcribed genes and regions of rapid histone turnover that are typically bound by the RSC chromatin-remodeling complex. Investigation of chromosome folding in mutants confirms roles for RSC, "gene looping" factor Ssu72, Mediator, H3K56 acetyltransferase Rtt109, and the N-terminal tail of H4 in folding of the yeast genome. This approach provides detailed structural maps of a eukaryotic genome, and our findings provide insights into the machinery underlying chromosome compaction.
Copyright © 2015 Elsevier Inc. All rights reserved.
Figures
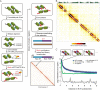
(A) Overview of the Micro-C method. Bottom right panel shows Micro-C data for yeast chromosome 6. (B) Zoom-in on a 20 kb × 20 kb submatrix from chromosome 9 (360,001-380,000), with Micro-C interactions represented in white-yellow-red-black heatmap showing the interaction intensity for a given pair of loci. (C) Decay of internucleosomal interactions with distance. Distances along the x axis are provided in units of nucleosomes – first data point represents ligation between adjacent (N/N+1) nucleosomes, with data out to N/N+60 products shown. Y axis shows log2 of the number of ligation products, normalized to parts per million (for interactions out to 100 kb) for each dataset. Data for 20 wild-type replicates, and for no crosslinking and no ligation control datasets, are indicated. Schematics illustrate nucleosomes contributing to N/N+1, N/N+2, and N/N+3 ligation products, using a tetranucleosome cartoon for illustration. See also Supplemental Figures S1-S2.
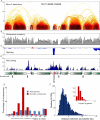
(A-D) Example of boundary identification. Data for a 15 kb locus, with arcs showing interactions between nucleosomes, colored as in Figure 1B. Interactions observed only once in the entire dataset have been removed for clarity. Gene annotations for this locus, and boundary calls shown in black arrows, are shown below panel (D). Emphasis on RPS26A shows both the overall lack of local Micro-C interactions, as well as the unusually strong boundary activity associated with this highly-transcribed gene. RNA-Seq data (B), nucleosome positioning data (C) and Sth1 ChIP-Seq enrichment (Lopez-Serra et al., 2014) (D) are also shown for this locus to emphasize the correlation between RSC-enriched promoters and boundary activity. (E) Boundaries between CIDs occur at promoters. For each nucleosome position relative to a gene, the fraction of boundary nucleosomes, or of all nucleosomes genome-wide, is shown on the y axis. As boundaries as defined here fall between adjacent nucleosomes, we show data here for the downstream boundary nucleosome, relative to underlying gene orientation – upstream nucleosomes are correspondingly enriched for −1 nucleosomes (and +N nucleosomes). (F) Length distribution of CIDs. Distribution of distances between boundary nucleosomes is plotted in blue using base pairs for the x axis, and in the inset using gene count as the scale. See also Supplemental Figures S3-S4.
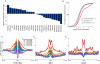
(A) Average histone modification levels are shown for all boundary nucleosomes (using both nucleosomes flanking a boundary). Histone modification data are from (Weiner et al., 2015), normalized to nucleosome occupancy, and expressed as log2 enrichment relative to all nucleosomes genome-wide. (B) Boundary activity at promoters is associated with elevated transcription rates. For each promoter, the transcription rate is defined as the Pol2 level (Kim et al., 2010) of the more highly-transcribed of the two adjacent genes, and cumulative plots show Pol2 enrichment values for nonboundary promoters, for all boundary promoters, and for the strongest half of boundary promoters. (C-E) Boundary nucleosome-depleted regions (NDRs) are associated with high levels of H3K18ac, RSC, and cohesin relative to all NDRs genome-wide. In each panel, all NDRs in the yeast genome were identified, and Micro-C interactions that cross the NDR were calculated. NDRs were sorted according to quintiles based on the abundance of NDR-spanning Micro-C reads – the first quintile encompasses the 1300 NDRs with the fewest NDR-spanning Micro-C ligation products, etc. – and ChIP-Seq data for the indicated factors was averaged for each quintile. See also Supplemental Figure S5.
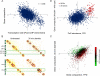
(A) Scatterplot comparison of gene compaction with transcription rate. X axis shows Pol2 ChIP data from (Kim et al., 2010), y axis shows gene compaction from this dataset based specifically on interactions beyond N/N+3, normalized for nucleosome occupancy and gene length (Supplemental Figures S6A-D). (B) Changes in transcription affect gene compaction. Micro-C was carried out for yeast subject to 30 minutes of 1.5 mM diamide, a sulfhydryl-reducing agent that alters transcription of ~20% of the yeast genome (Gasch et al., 2000). Here, Micro-C contact matrices for unstressed and diamide-stressed yeast show regions surrounding three ribosomal protein-encoding genes (RPGs), which are strongly repressed in response to diamide stress and which exhibit a dramatic increase in local compaction. (C) Systematic analysis of diamide-induced changes in chromosome folding. Here, gene compaction is scatterplotted for unstressed and diamide-stressed yeast, with points color-coded according to the corresponding mRNA abundance changes in diamide (smoothed by 20 nearest neighbors). (D) Global inhibition of transcription leads to increased compaction over normally highly-transcribed genes. Here, Pol2 abundance at t=0 is plotted (x axis) against the change in gene compaction in response to 45 minutes of treatment with the RNA polymerase inhibitor thiolutin. Red points show RPGs. See also Supplemental Figure S6.
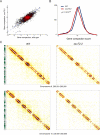
A) Micro-C was carried out for three replicate cultures of ssu72-2. Compaction scores for all genes are scatterplotted for wild-type and ssu72-2 mutants, as indicated. (B) Histogram of gene compaction scores for wild-type and ssu72-2 mutants, showing a subtle but significant (p < 1.2e-8, KS test) general loss of gene compaction in the mutant. (C) Two 20 kb × 20 kb matrixes showing wild type and ssu72-2 Micro-C data, as indicated. See also Supplemental Figure S6.
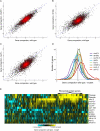
(A-C) Scatterplots of occupancy-corrected gene compaction scores for wild type (x axis), and the various indicated mutants. Notable here are a relatively tight scatterplot for rnh201Δ (A), a more variable signal with a modest global loss of compaction for rtt109Δ (B), and a dramatic loss of gene compaction for med1Δ (C). (D) Histograms showing the distribution of changes in gene compaction for the indicated mutants or conditions. In each case, nucleosome occupancy-corrected gene compaction was calculated for every gene in the genome, and the difference between BY4741 and the indicated mutants is plotted on the x axis – negative values indicate decreased gene compaction in the mutant, positive values indicate increased gene compaction. (E) Global and gene-specific effects of chromatin mutants on gene compaction. For all mutants analyzed by Micro-C, gene compaction scores were calculated, and for all genes with at least a 2-fold change in compaction in one mutant, the difference between all mutants and the relevant wild-type is shown in a clustered heatmap. See also Supplemental Figure S7.
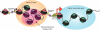
Cartoon showing a model for one relatively poorly-transcribed gene adjacent to a more open highly-transcribed gene.
Comment in
-
Filling the gap: Micro-C accesses the nucleosomal fiber at 100-1000 bp resolution.
Mozziconacci J, Koszul R. Mozziconacci J, et al. Genome Biol. 2015 Aug 21;16(1):169. doi: 10.1186/s13059-015-0744-8. Genome Biol. 2015. PMID: 26294274 Free PMC article.
-
Genomics. Micro-C maps of genome structure.
de Souza N. de Souza N. Nat Methods. 2015 Sep;12(9):812. doi: 10.1038/nmeth.3575. Nat Methods. 2015. PMID: 26554092 No abstract available.
Similar articles
-
Filling the gap: Micro-C accesses the nucleosomal fiber at 100-1000 bp resolution.
Mozziconacci J, Koszul R. Mozziconacci J, et al. Genome Biol. 2015 Aug 21;16(1):169. doi: 10.1186/s13059-015-0744-8. Genome Biol. 2015. PMID: 26294274 Free PMC article.
-
Micro-C XL: assaying chromosome conformation from the nucleosome to the entire genome.
Hsieh TS, Fudenberg G, Goloborodko A, Rando OJ. Hsieh TS, et al. Nat Methods. 2016 Dec;13(12):1009-1011. doi: 10.1038/nmeth.4025. Epub 2016 Oct 10. Nat Methods. 2016. PMID: 27723753
-
Nucleosome positions alone can be used to predict domains in yeast chromosomes.
Wiese O, Marenduzzo D, Brackley CA. Wiese O, et al. Proc Natl Acad Sci U S A. 2019 Aug 27;116(35):17307-17315. doi: 10.1073/pnas.1817829116. Epub 2019 Aug 15. Proc Natl Acad Sci U S A. 2019. PMID: 31416914 Free PMC article.
-
Yeast HMO1: Linker Histone Reinvented.
Panday A, Grove A. Panday A, et al. Microbiol Mol Biol Rev. 2016 Nov 30;81(1):e00037-16. doi: 10.1128/MMBR.00037-16. Print 2017 Mar. Microbiol Mol Biol Rev. 2016. PMID: 27903656 Free PMC article. Review.
-
Interplay among ATP-Dependent Chromatin Remodelers Determines Chromatin Organisation in Yeast.
Prajapati HK, Ocampo J, Clark DJ. Prajapati HK, et al. Biology (Basel). 2020 Jul 25;9(8):190. doi: 10.3390/biology9080190. Biology (Basel). 2020. PMID: 32722483 Free PMC article. Review.
Cited by
-
The chromatin tapestry as a framework for neurodevelopment.
Nolan B, Reznicek TE, Cummings CT, Rowley MJ. Nolan B, et al. Genome Res. 2024 Oct 29;34(10):1477-1486. doi: 10.1101/gr.278408.123. Genome Res. 2024. PMID: 39472026 Free PMC article. Review.
-
dcHiC detects differential compartments across multiple Hi-C datasets.
Chakraborty A, Wang JG, Ay F. Chakraborty A, et al. Nat Commun. 2022 Nov 11;13(1):6827. doi: 10.1038/s41467-022-34626-6. Nat Commun. 2022. PMID: 36369226 Free PMC article.
-
Chromosome conformation capture technologies and their impact in understanding genome function.
Sati S, Cavalli G. Sati S, et al. Chromosoma. 2017 Feb;126(1):33-44. doi: 10.1007/s00412-016-0593-6. Epub 2016 Apr 30. Chromosoma. 2017. PMID: 27130552 Review.
-
Hi-CO: 3D genome structure analysis with nucleosome resolution.
Ohno M, Ando T, Priest DG, Taniguchi Y. Ohno M, et al. Nat Protoc. 2021 Jul;16(7):3439-3469. doi: 10.1038/s41596-021-00543-z. Epub 2021 May 28. Nat Protoc. 2021. PMID: 34050337
-
Mulet-Lazaro R, Delwel R. Mulet-Lazaro R, et al. Hemasphere. 2023 Nov 8;7(11):e969. doi: 10.1097/HS9.0000000000000969. eCollection 2023 Nov. Hemasphere. 2023. PMID: 37953829 Free PMC article. Review.
References
-
- Dekker J, Rippe K, Dekker M, Kleckner N. Capturing chromosome conformation. Science (New York, NY. 2002;295:1306–1311. - PubMed
Publication types
MeSH terms
Substances
Grants and funding
LinkOut - more resources
Full Text Sources
Other Literature Sources
Molecular Biology Databases