Daunorubicin-Loaded DNA Origami Nanostructures Circumvent Drug-Resistance Mechanisms in a Leukemia Model - PubMed
- ️Fri Jan 01 2016
Daunorubicin-Loaded DNA Origami Nanostructures Circumvent Drug-Resistance Mechanisms in a Leukemia Model
Patrick D Halley et al. Small. 2016.
Abstract
Many cancers show primary or acquired drug resistance due to the overexpression of efflux pumps. A novel mechanism to circumvent this is to integrate drugs, such as anthracycline antibiotics, with nanoparticle delivery vehicles that can bypass intrinsic tumor drug-resistance mechanisms. DNA nanoparticles serve as an efficient binding platform for intercalating drugs (e.g., anthracyclines doxorubicin and daunorubicin, which are widely used to treat acute leukemias) and enable precise structure design and chemical modifications, for example, for incorporating targeting capabilities. Here, DNA nanostructures are utilized to circumvent daunorubicin drug resistance at clinically relevant doses in a leukemia cell line model. The fabrication of a rod-like DNA origami drug carrier is reported that can be controllably loaded with daunorubicin. It is further directly verified that nanostructure-mediated daunorubicin delivery leads to increased drug entry and retention in cells relative to free daunorubicin at equal concentrations, which yields significantly enhanced drug efficacy. Our results indicate that DNA origami nanostructures can circumvent efflux-pump-mediated drug resistance in leukemia cells at clinically relevant drug concentrations and provide a robust DNA nanostructure design that could be implemented in a wide range of cellular applications due to its remarkably fast self-assembly (≈5 min) and excellent stability in cell culture conditions.
Keywords: DNA nanotechnology; DNA origami; drug delivery; drug resistance; leukemia.
© 2015 WILEY-VCH Verlag GmbH & Co. KGaA, Weinheim.
Figures
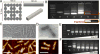
Design and construction of a DNA origami engineered rod-like Horse nanostructure drug delivery system. (A) 2- and 3-dimensional design schematics of the Horse nanostructure (~92.5 × ~13.2 × ~11 nm) containing four open cavities that extend along the length of the structure with a total surface area (~8787.5nm2). (B) Upon completion of a 17-hour DNA origami molecular self-assembly reaction, samples were resolved via agarose gel electrophoresis (left to right; L = DNA ladder, S = 7249 M13mp18 scaffold, 1 = Horse DNA nanostructure, 2 = Horse nanostructure with 10 overhangs, 3 = Horse nanostructure with 36 overhangs, 4 = Yoyo-1-labeled Horse nanostructure, and 5 = Horse DNA nanostructures loaded with daunorubicin to a base pair binding ratio of 0.42). The inset shows a brightened image of lanes 4 and 5. (C) Leading DNA bands were excised from agarose gels, gel purified and imaged by transmission electron microscopy (TEM, top) and atomic force microscopy (AFM, bottom). (D) Daunorubicin loaded Horse DNA nanostructures at a base pair binding ratio of ~1 were imaged via TEM (top) and AFM (bottom) revealing fraying of the structure upon drug loading. Representative gels and TEM images are shown from three independent experiments. (E) Horse DNA nanostructures were mixed with complete RPMI 1640 medium supplemented with 20% FBS at 37°C for 3, 6, 9, 12, 15, and hours to address stability via agarose gel electrophoresis. (F) Horse DNA nanostructure folding reactions were subjected to a ‘rapid fold’ thermal annealing ramp for 1, 5, 15, 30, 60, and 120 minutes and evaluated via agarose gel electrophoresis. Representative gels are shown from two independent experiments.
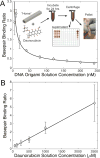
Daunorubicin effectively intercalates and releases from the Horse DNA nanostructure. (A) Varying concentrations of Horse DNA nanostructures (5–240 nM) were added to 500 μM daunorubicin, and the amount of loaded daunorubicin was determined colorimetrically by absorbance. The inset illustrates the process of drug loading including mixing, incubation, centrifugation, and measuring the daunorubicin concentration left in the supernatant. The data are presented in triplicate as mean bp binding ratio ± SD (SD was smaller than the marker size for larger concentrations). (B) Varying concentrations of daunorubicin (62.5–2500 μM) were added to Horse nanostructures at 20 nM for 24 hours followed by free daunorubicin removal. Linear regression statistical analysis was applied to the data set. The data are presented as normalized mean relative intensity ± SD in triplicate and represent three independent experiments.
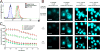
Daunorubicin-loaded Horse DNA nanostructures are internalized by HL-60/ADR cells. (A) Either free daunorubicin or daunorubicin-loaded Horse DNA nanostructures (1 μM daunorubicin) were introduced to HL-60/ADR cells for 24 hours. The level of intracellular daunorubicin fluorescence was determined via flow cytometry. Cells were considered viable using Near IR LIVE/DEAD viability cell stain. A representative histogram overlay is shown from three independent experiments. (B) Either free daunorubicin or daunorubicin loaded Horse DNA nanostructures (1 μM daunorubicin) were introduced to HL-60/ADR or HL-60 parental cells for three hours followed by a PBS wash and subject to live cell fluorescence imaging via total internal reflection (TIRF) (488 nm excitation) at ten minute intervals for approximately three hours. Scale bars are 10 μm. (C) Time traces of the level of intracellular daunorubicin were measured from at least 50 individual cells where the data are presented as the mean relative fluorescent unit (RFU) ± SEM where data and images represent two independent experiments.
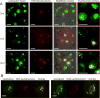
Visualization of Horse DNA nanostructure entry and lysosomal compartment localization in HL-60/ADR cells to induce cell death. (A) Cy3-labeled Horse DNA nanostructures (561 nm excitation) and Lysotracker Green fluorescent molecules (488 nm excitation) were added to HL-60/ADR cells and monitored via epifluorescence and bright field (DIC) time lapse imaging from 2 to 18 hours after incubation of nanostructures. Representative images are shown at 3, 9, and 15 hours post nanostructure addition from three independent experiments. The first column depicts acidic lysosomes, the second column depicts Cy3-labeled Horse nanostructures, the third column represents a DIC, 488 nm and 561nm channel overlay, and the fourth column shows zoomed-in overlays of the dashed red box showing acidic compartments (green), Horse nanostructures (red) and co-localized regions (yellow). (B) Alexa647-labeled Horse DNA nanostructures (640 nm excitation) and Lysotracker Green fluorescent molecules (488 nm excitation) were added to HL-60/ADR cells for 1 hour and visualized via confocal microscopy. The first column depicts acidic lysosomes, the second column depicts Alexa647-labeled Horse nanostructures, the third column represents a 488 nm and 640 nm channel overlay Representative merged images are shown at varying time points from three independent experiments. Scale bars are 10 μm in (A) and 5 μm in (B).
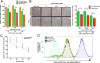
Daunorubicin-loaded Horse DNA nanostructures circumvent drug resistance in HL-60/ADR cells. (A) Varying concentrations of free daunorubicin (0.1, 0.25, and 1 μM), unloaded Horse nanostructures, or daunorubicin-loaded Horse DNA nanostructures (BPBR of ~0.9) were added to HL-60/ADR cells and cell viability was evaluated after 24 hours using the CCK-8 assay. Statistical differences are shown between groups. *, p < 0.05 (0.1 μM, p < 0.05, 95% CI: 0.3840 to 37.52; 0.25 μM, p < 0.05, 95% CI: 0.9718 to 37.36; 1.0 μM, p < 0.05, 95% CI: 0.1861 to 38.19). (B) Varying concentrations (0.1, 0.25, and 1 μM) of free daunorubicin or daunorubicin-loaded Horse nanostructures were added to HL-60/ADR cells for 24 hours and the number of viable cells and relative density (indicated by n = number of viable cells) was evaluated using fluorescence microscopy using the viability dye, Sytox Red, with 561 nm laser excitation. The image shows a representative DIC image overlaid with the Sytox Red fluorescence image. Statistical differences are shown between groups. *, p < 0.05 (0.1 μM, p = 0.0423, 95% CI: 0.9487 to 38.35; 0.25 μM, p = 0.0104, 95% CI: 9.979 to 41.09; 1.0 μM, p = 0.0230, 95% CI: 3.057 to 28.60). (C) Either free daunorubicin or daunorubicin-loaded Horse nanostructures (1 μM daunorubicin) were added to HL-60/ADR cells for the first 24 hour over a four day time course while the cells were washed with PBS at 24 and 72 hours post initial treatment. The relative number of viable cells was determined via counting beads via flow cytometry. The mean number of viable cells ± SEM are shown at 24, 48, 72, and 96 hours post initial nanostructure addition. Statistical differences are shown between groups. *, p < 0.05 (24 hours, p = 0.0151, 95% CI: 3.144 to 19.89; 48 hours, p = 0.0492, 95% CI: 0.1682 to 60.32). (D) Either free daunorubicin or daunorubicin-loaded Horse nanostructures (1 μM daunorubicin) were added to HL-60/ADR cells over a four day time course while the cells were washed with PBS at 24 and 72 hours post initial treatment. The near IR live/dead viability stain and the Violet V450 proliferation dye was added to evaluate proliferation via flow cytometry. Cells negative for Near IR LIVE/DEAD viability stain were gated and considered viable. Proliferation was evaluated via V450 proliferation dye fluorescence represented by a representative histogram overlay at 96 hours from three independent experiments. The quantitative data in A, B, and C were normalized to cells only controls and are presented in triplicate as the mean relative % (or relative number, C) viable cells ± SEM and represent three independent experiments.
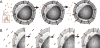
Daunorubicin-loaded Horse DNA nanostructure drug delivery system proposed model. (A) Free drug enters the cell by passive diffusion across the membrane and is quickly expelled from drug resistance HL-60/ADR cells via efflux pumps. (B) In contrast, daunorubicin-loaded Horse DNA nanostructure are endocytosed and enter the endolyosomal pathway, thus circumventing the MRP-1 efflux pumps expressed on the cell surface. The acidic environment of the endolysosome (and possibly lower ion concentrations and the presentence of nucleases) facilitates daunorubicin release that can passively diffuse into the cytoplasm. The large level of free daunorubicin molecules is then available to enter the nucleus to disrupt DNA replication to ultimately impair cellular growth.
Similar articles
-
DNA origami as a carrier for circumvention of drug resistance.
Jiang Q, Song C, Nangreave J, Liu X, Lin L, Qiu D, Wang ZG, Zou G, Liang X, Yan H, Ding B. Jiang Q, et al. J Am Chem Soc. 2012 Aug 15;134(32):13396-403. doi: 10.1021/ja304263n. Epub 2012 Aug 1. J Am Chem Soc. 2012. PMID: 22803823
-
Qu X, Wan C, Becker HC, Zhong D, Zewail AH. Qu X, et al. Proc Natl Acad Sci U S A. 2001 Dec 4;98(25):14212-7. doi: 10.1073/pnas.241509698. Epub 2001 Nov 27. Proc Natl Acad Sci U S A. 2001. PMID: 11724924 Free PMC article.
-
Jin J, Pastrello D, Penning NA, Jones AT. Jin J, et al. Int J Biochem Cell Biol. 2008;40(10):2240-52. doi: 10.1016/j.biocel.2008.03.004. Epub 2008 Mar 16. Int J Biochem Cell Biol. 2008. PMID: 18439867
-
Daunorubicin and doxorubicin, anthracycline antibiotics, a physicochemical and biological review.
Aubel-Sadron G, Londos-Gagliardi D. Aubel-Sadron G, et al. Biochimie. 1984 May;66(5):333-52. doi: 10.1016/0300-9084(84)90018-x. Biochimie. 1984. PMID: 6380596 Review.
-
DNA Nanostructures as Smart Drug-Delivery Vehicles and Molecular Devices.
Linko V, Ora A, Kostiainen MA. Linko V, et al. Trends Biotechnol. 2015 Oct;33(10):586-594. doi: 10.1016/j.tibtech.2015.08.001. Trends Biotechnol. 2015. PMID: 26409777 Review.
Cited by
-
Ijäs H, Shen B, Heuer-Jungemann A, Keller A, Kostiainen MA, Liedl T, Ihalainen JA, Linko V. Ijäs H, et al. Nucleic Acids Res. 2021 Apr 6;49(6):3048-3062. doi: 10.1093/nar/gkab097. Nucleic Acids Res. 2021. PMID: 33660776 Free PMC article.
-
Liu Y, Yu S, Chen Y, Hu Z, Fan L, Liang G. Liu Y, et al. Front Pharmacol. 2024 Apr 16;15:1376955. doi: 10.3389/fphar.2024.1376955. eCollection 2024. Front Pharmacol. 2024. PMID: 38689664 Free PMC article. Review.
-
CAGE: Chromatin Analogous Gene Expression.
Zadegan RM, Hughes WL. Zadegan RM, et al. ACS Synth Biol. 2017 Oct 20;6(10):1800-1806. doi: 10.1021/acssynbio.7b00045. Epub 2017 Jun 30. ACS Synth Biol. 2017. PMID: 28657718 Free PMC article.
-
Rationally Designed DNA Nanostructures for Drug Delivery.
Xu F, Xia Q, Wang P. Xu F, et al. Front Chem. 2020 Sep 24;8:751. doi: 10.3389/fchem.2020.00751. eCollection 2020. Front Chem. 2020. PMID: 33195016 Free PMC article. Review.
-
Rationally Programming Nanomaterials with DNA for Biomedical Applications.
He L, Mu J, Gang O, Chen X. He L, et al. Adv Sci (Weinh). 2021 Feb 24;8(8):2003775. doi: 10.1002/advs.202003775. eCollection 2021 Apr. Adv Sci (Weinh). 2021. PMID: 33898180 Free PMC article. Review.
References
-
- Michieli M, Damiani D, Ermacora A, Geromin A, Michelutti A, Masolini P, Baccarani M. British journal of haematology. 2000;108(4):703–9. - PubMed
-
- Hofmeister CC, Yang X, Pichiorri F, Chen P, Rozewski DM, Johnson AJ, Lee S, Liu Z, Garr CL, Hade EM, Ji J, Schaaf LJ, Benson DM, Jr, Kraut EH, Hicks WJ, Chan KK, Chen CS, Farag SS, Grever MR, Byrd JC, Phelps MA. Journal of clinical oncology: official journal of the American Society of Clinical Oncology. 2011;29(25):3427–34. doi: 10.1200/JCO.2010.32.4962. - DOI - PMC - PubMed
Publication types
MeSH terms
Substances
Grants and funding
LinkOut - more resources
Full Text Sources
Other Literature Sources
Medical