DNA sliding in nucleosomes via twist defect propagation revealed by molecular simulations - PubMed
- ️Mon Jan 01 2018
DNA sliding in nucleosomes via twist defect propagation revealed by molecular simulations
Giovanni B Brandani et al. Nucleic Acids Res. 2018.
Abstract
While nucleosomes are highly stable structures as fundamental units of chromatin, they also slide along the DNA, either spontaneously or by active remodelers. Here, we investigate the microscopic mechanisms of nucleosome sliding by multiscale molecular simulations, characterizing how the screw-like motion of DNA proceeds via the formation and propagation of twist defects. Firstly, coarse-grained molecular simulations reveal that the sliding dynamics is highly dependent on DNA sequence. Depending on the sequence and the nucleosome super-helical location, we find two distinct types of twist defects: a locally under-twisted DNA region, previously observed in crystal structures, and a locally over-twisted DNA, an unprecedented feature. The stability of the over-twist defect was confirmed via all-atom simulations. Analysis of our trajectories via Markov state modeling highlights how the sequence-dependence of the sliding dynamics is due to the different twist defect energy costs, and in particular how nucleosome regions where defects cannot easily form introduce the kinetic bottlenecks slowing down repositioning. Twist defects can also mediate sliding of nucleosomes made with strong positioning sequences, albeit at a much lower diffusion coefficient, due to a high-energy intermediate state. Finally, we discuss how chromatin remodelers may exploit these spontaneous fluctuations to induce unidirectional sliding of nucleosomes.
Figures
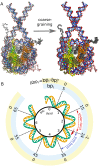
Coarse-graining and defect formation. (A) All-atom (on the left) and coarse-grained (right) nucleosome structures. Histones H3 in light and dark gray, H4 in orange and light green, H2A in yellow and white, and H2B in dark green and pink. For the DNA, phosphate groups are in red, sugar groups in cyan and bases in blue. (B) Schematics of a half nucleosome structure, mechanism of defect formation and analysis method. Super-helical locations (SHLs) start from 0 at the dyad and increase by 1 every DNA turn. histone–DNA contact points are at the half-integer SHLs. For each configuration in the MD trajectories, we track the base pair indexes bpi at the 14 histone–DNA contact points, and their value relative to the initial one observed in the 1KX5 crystal structure configuration, which we refer to as the contact indexes Δbpi = bpi – bpiX. At the beginning, when no twist defects are present, all contact indexes will be zero. If, for instance, after some time the DNA undergoes a clockwise screw-like motion by 1 base pair at contact point 2.5, then the DNA segment at SHL 2 will have a missing base pair relative to the initial structure, whereas at SHL 3 there will be an extra base pair, corresponding respectively to under-twist and over-twist defects. These defects can be quantified by the difference k between two neighboring contact indexes (respectively k = –1 and k = +1 in the example).
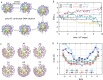
Screw-like sliding of DNA. (A) Representative snapshots from a poly-AC trajectory (from 8.5 × 106 to 9.5 × 106 MD steps in panel B). During DNA sliding, the overall DNA path remains close to the initial crystal structure configuration. Two reference phosphates initially at the dyad are represented as red spheres, highlighting the screw-like motion of DNA. For the purpose of clarity, we only depict the central 70 bp of DNA. The histone color scheme is identical to that in Figure 1. (B) The dynamics of contact index 0.5 (next to the dyad) for representative trajectories of the six considered sequences, showing that we observe repositioning by a few base pairs within the considered time scales. (C) In pink, overlay of all DNA backbone conformations observed during the MD simulations shown in panel B. For comparison, we show in blue the DNA path in the 1KX5 crystal structure. For clarity, we only depict half of the nucleosome, from SHL = 0 to SHL = 7 (the other half of the nucleosome shows a similar behavior). (D) As a function of SHL, the average root mean square deviation from the reference 1KX5 structure of the nucleosomal regions corresponding to the 14 contact points at the half-integer SHLs.
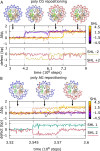
Repositioning via defect propagation. Representative fragments of trajectories for the poly-CG and AG sequences, in panels A and B respectively. On the top (for each panel), nucleosome conformations during repositioning: the DNA path is initially colored in blue, and when a phosphate group moves by 1 bp in the negative SHL direction (clockwise) along the DNA backbone, we change the color of the nucleotide to red. On the center, dynamics of histone–DNA contact indexes from SHL –4.5 to 4.5 for sections of the representative trajectories. On the bottom, dynamics of the defect variables k at the SHLs where stable defects are formed.
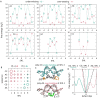
Free energy and structure of defects. (A) Free energy costs of DNA under-twisting (cyan circles) and over-twisting (pink crosses) as a function of sequence and super-helical location. For poly-AA and poly-CC sequences, we only show the results for the five central SHLs, because of the large fluctuations further away from the dyad. (B) Summary of the probabilities of under-twisting and over-twisting (proportional to the circle areas, in cyan and pink respectively) for each DNA sequence and SHL (from 0 to 5, negatives SHLs show similar behavior). Ordering is based on the propensity of the two defect types. (C) Examples of defect structures observed during our simulations: under-twisting at SHL –2 in poly-CG nucleosomes (in cyan), compared to the standard crystal structure form (black), and over-twisting at SHL 1 in poly-AG (pink), again compared to the standard form. Phosphate groups are highlighted as spheres. The missing or extra base pair is accommodated via deformations on the outer side of the DNA lacking contacts with the histones (partially shown following the color scheme of the other figures). Each DNA structure has been obtained by averaging over 100 MD frames from the representative trajectories shown in Figure 3. (D) Examples of potentials of mean force along the defect coordinates k for three representative sequences and SHLs: poly-CG at SHL 2 (cyan, solid line), where a metastable under-twist defect can form; poly-CG at SHL 3 (black, dotted line), with a unimodal distribution highlighting the absence of stable defects; poly-AG at SHL 1 (pink, dashed line), where over-twisting is metastable.
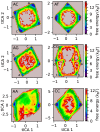
Free-energy surfaces. For each 2-bp periodic DNA sequence, we show the free energy landscapes obtained via Markov state modeling along the first and second tICA coordinates. Each local minimum correspond to a long-lived metastable basin of the system (see Supplementary Figures S6 and S7). The typical nucleosome conformations and defect patterns observed in some of the basins (labeled by a, b, c or d in poly-AC, AG and CG) are represented via a set of cartoons in Figure 6.
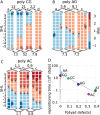
Sliding pathways and kinetics derived from Markov state models. (A–C) MSMs for poly-CG, AG, and AC (which is similar to the omitted AT case) in panels A, B and C, respectively, showing the mean first passage times (MFPTs) of the transitions between the long-lived basins of the system along a 1 bp repositioning pathway, and a sample of the most populated nucleosome conformations within each basin. The labels (a, b, c and d) on the states correspond to those indicated in the free energy landscapes of Figure 5. Each column represents a nucleosome conformation, with each colored square representing a section of 10 bps of DNA bound to a nucleosome contact point. The color of the square correspond to the contact index of that DNA section, with the DNA undergoing a screw-like rotation in the negative SHL direction as the color changes from blue towards red. The presence of under-twisting or over-twisting at each SHL is respectively indicated by the symbols – and +. MFPTs are given near the arrows in units of 106 MD steps. Most basins have similar equilibrium probabilities, except for the poly-CG case, where the basins with a defect at SHL ±2 have a free energy of about 2kBT relative the basins without defects there. (D) Repositioning times for each DNA sequence as a function of the average probability to find excited defect configurations at the three central SHLs (–1,0,1). Here we consider any deviation from the lowest energy state, which in most cases correspond to the configuration found in the 1KX5 reference. However, in the case of poly-CC, over-twist defects are the ground state at SHL ±1, and we consider instead the probability to find a non-defect (excited) state, which is expected to be relevant for repositioning.
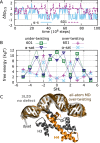
Stability and defect formation in positioning sequences. (A) The dynamics of contact index 0.5 for 601 and α-satellite nucleosomes for two representative trajectories, starting from the optimal conformations found in the respective crystal structures. (B) The free-energy costs of defect formation at each SHL for 601 and α-satellite positioning sequences. (C) Structure of 601 nucleosomes around SHL –1, comparing the crystal structure with PDB id 3LZ0 (gray transparency) to the metastable form with over-twisting (brown). For the latter, we show 10 DNA frames sampled from our 100-ns all-atom MD simulation starting from a configuration back-mapped from a coarse-grained trajectory. We also show the region of the H3H4 tetramer involved in the interactions with the DNA, highlighting that the extra base pair is accommodated in the outer DNA region lacking histone contacts.
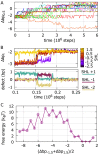
Repositioning of 601 nucleosomes via defect formation. (A) Dynamics of contact index 0.5 for a sample of 20 trajectories starting from an initial configuration shifted by 3 bp from the crystal structure. In most trajectories, repositioning proceeds toward the optimal crystal structure configuration with contact indexes zero; with this shifted initial condition, out of a total of 400 MD runs, we only find 3 sliding events going in the opposite direction. (B) Dynamics of the central contact indexes during a section of a sliding trajectory (top), showing that repositioning occurs via formation or disappearance of defects at SHLs –2, –1 and +1 (bottom). (C) Free energy profile of 601 repositioning reconstructed via Markov state modeling, as a function of the average of the contact indexes at SHLs ±1.5. Integer states have no defects, while half-integer ones have DNA over-twisting at SHLs 0, –1 or +1.
Similar articles
-
Chromatin remodelers couple inchworm motion with twist-defect formation to slide nucleosomal DNA.
Brandani GB, Takada S. Brandani GB, et al. PLoS Comput Biol. 2018 Nov 5;14(11):e1006512. doi: 10.1371/journal.pcbi.1006512. eCollection 2018 Nov. PLoS Comput Biol. 2018. PMID: 30395604 Free PMC article.
-
The dynamics of the nucleosome: thermal effects, external forces and ATP.
Blossey R, Schiessel H. Blossey R, et al. FEBS J. 2011 Oct;278(19):3619-32. doi: 10.1111/j.1742-4658.2011.08283.x. Epub 2011 Sep 2. FEBS J. 2011. PMID: 21812931 Review.
-
Aoyagi S, Wade PA, Hayes JJ. Aoyagi S, et al. J Biol Chem. 2003 Aug 15;278(33):30562-8. doi: 10.1074/jbc.M304148200. Epub 2003 May 26. J Biol Chem. 2003. PMID: 12767978
-
Chromatin dynamics: nucleosomes go mobile through twist defects.
Kulić IM, Schiessel H. Kulić IM, et al. Phys Rev Lett. 2003 Oct 3;91(14):148103. doi: 10.1103/PhysRevLett.91.148103. Epub 2003 Oct 1. Phys Rev Lett. 2003. PMID: 14611559
-
Mechanisms of ATP-dependent nucleosome sliding.
Bowman GD. Bowman GD. Curr Opin Struct Biol. 2010 Feb;20(1):73-81. doi: 10.1016/j.sbi.2009.12.002. Epub 2010 Jan 8. Curr Opin Struct Biol. 2010. PMID: 20060707 Free PMC article. Review.
Cited by
-
Session 1SEA-physics of chromatin dynamics at the 57th Biophysical Society of Japan meeting.
Ito Y, Kimura A. Ito Y, et al. Biophys Rev. 2020 Apr;12(2):265-266. doi: 10.1007/s12551-020-00642-3. Epub 2020 Feb 13. Biophys Rev. 2020. PMID: 32056110 Free PMC article. No abstract available.
-
Surprising Twists in Nucleosomal DNA with Implication for Higher-order Folding.
Todolli S, Young RT, Watkins AS, Bu Sha A, Yager J, Olson WK. Todolli S, et al. J Mol Biol. 2021 Sep 3;433(18):167121. doi: 10.1016/j.jmb.2021.167121. Epub 2021 Jun 28. J Mol Biol. 2021. PMID: 34192585 Free PMC article.
-
The kinetic landscape of nucleosome assembly: A coarse-grained molecular dynamics study.
Brandani GB, Tan C, Takada S. Brandani GB, et al. PLoS Comput Biol. 2021 Jul 27;17(7):e1009253. doi: 10.1371/journal.pcbi.1009253. eCollection 2021 Jul. PLoS Comput Biol. 2021. PMID: 34314440 Free PMC article.
-
ssDNA diffuses along replication protein A via a reptation mechanism.
Mishra G, Bigman LS, Levy Y. Mishra G, et al. Nucleic Acids Res. 2020 Feb 28;48(4):1701-1714. doi: 10.1093/nar/gkz1202. Nucleic Acids Res. 2020. PMID: 31919510 Free PMC article.
-
Bendandi A, Dante S, Zia SR, Diaspro A, Rocchia W. Bendandi A, et al. Front Mol Biosci. 2020 Feb 25;7:15. doi: 10.3389/fmolb.2020.00015. eCollection 2020. Front Mol Biosci. 2020. PMID: 32158765 Free PMC article. Review.
References
-
- Richmond T.J., Davey C.A.. The structure of DNA in the nucleosome core. Nature. 2003; 423:145–150. - PubMed
-
- van Holde K.E. Chromatin. 1989; NY: Springer.
-
- Freeman G.S., Lequieu J.P., Hinckley D.M., Whitmer J.K., de Pablo J.J.. DNA shape dominates sequence affinity in nucleosome formation. Phys. Rev. Lett. 2014; 113:168101. - PubMed
Publication types
MeSH terms
Substances
LinkOut - more resources
Full Text Sources
Other Literature Sources