Green function of correlated genes in a minimal mechanical model of protein evolution - PubMed
- ️Mon Jan 01 2018
Green function of correlated genes in a minimal mechanical model of protein evolution
Sandipan Dutta et al. Proc Natl Acad Sci U S A. 2018.
Abstract
The function of proteins arises from cooperative interactions and rearrangements of their amino acids, which exhibit large-scale dynamical modes. Long-range correlations have also been revealed in protein sequences, and this has motivated the search for physical links between the observed genetic and dynamic cooperativity. We outline here a simplified theory of protein, which relates sequence correlations to physical interactions and to the emergence of mechanical function. Our protein is modeled as a strongly coupled amino acid network with interactions and motions that are captured by the mechanical propagator, the Green function. The propagator describes how the gene determines the connectivity of the amino acids and thereby, the transmission of forces. Mutations introduce localized perturbations to the propagator that scatter the force field. The emergence of function is manifested by a topological transition when a band of such perturbations divides the protein into subdomains. We find that epistasis-the interaction among mutations in the gene-is related to the nonlinearity of the Green function, which can be interpreted as a sum over multiple scattering paths. We apply this mechanical framework to simulations of protein evolution and observe long-range epistasis, which facilitates collective functional modes.
Keywords: Green function; dimensional reduction; epistasis; genotype-to-phenotype map; protein evolution.
Copyright © 2018 the Author(s). Published by PNAS.
Conflict of interest statement
The authors declare no conflict of interest.
Figures
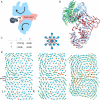
Protein as an evolving machine and propagation of mechanical forces. (A) Formation of a softer shear band (red) separating the protein into two rigid subdomains (light blue). When a ligand binds, the biochemical function involves a low-energy hinge-like or shear motion (arrows). (B) Shear band and large-scale motion in a real protein: the arrows show the displacement of all amino acids in human glucokinase when it binds glucose (Protein Data Bank ID codes 1v4s and 1v4t). The coloring shows a high-shear region (red) separating two low-shear domains that move as rigid bodies (shear calculated as in refs. and 36). (C) The mechanical model. The protein is made of two species of amino acids, polar (P; red) and hydrophobic (H; blue), with a sequence that is encoded in a gene. Each amino acid forms weak or strong bonds with its 12 near neighbors (Right) according to the interaction rule in the table (Left). (D) The protein is made of 10×20=200 amino acids with positions that are randomized from a regular triangular lattice. Strong bonds are shown as gray lines. Evolution begins from a random configuration (Left) and evolves by mutating one amino acid at each step, switching between H and P. The fitness is the mechanical response to a localized force probe (pinch) (2). After ∼103 mutations (Center; intermediate stage), the evolution reaches a solution (Right). The green arrows show the mechanical response: a hinge-like, low-energy motion with a shear band starting at the probe and traversing the protein, qualitatively similar to B. L, left; R, right.
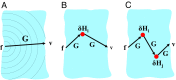
Force propagation, mutations, and epistasis. (A) The Green function G measures the propagation of the mechanical signal, depicted as a “diffraction wave,” across the protein (blue) from the force source f (pinch) to the response site v. (B) A mutation δHi deflects the propagation of force. The effect of the mutation on the propagator δG can be described as a series of multiple scattering paths (6). (C) The epistasis between two mutations, δHi and δHj, is equivalent to a series of multiple scattering paths (9).
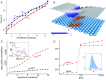
The mechanical Green function and the emergence of protein function. (A) Progression of the fitness F during the evolution run shown in Fig. 1D (black) together with the fitness trajectory averaged over ∼106 runs ⟨F⟩ (red). Shown are the last 16 beneficial mutations toward the formation of the channel. The contribution of the emergent low-energy mode ⟨F*⟩ (blue) dominates the fitness [4]. (B) Landscape of the fitness change δF [3] averaged over ∼106 solutions for all 200 possible positions of point mutations at a solution. Underneath, the average amino acid configuration of the protein is shown in shades of red (P) and blue (H). In most sites, mutations are neutral, while mutations in the channel are deleterious on average. L, left. (C) The average magnitude of the two-codon correlation |Qij| [5] in the shear band (amino acids in rows 7–13; red) and in the whole protein (black) as a function of the number of beneficial mutations, t. (Inset) Profile of the spatial correlation g(r) within the shear band (after t=1,11,16 beneficial mutations). (D) The mean shear in the protein in a single run (black) and averaged over ∼106 solutions (red) as a function of the fraction of P amino acids, p. The values of p are shifted by the position of the jump, pc. (Inset) Distribution of pc.
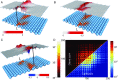
Mechanical epistasis. The epistasis [7], averaged over ∼106 solutions Eij=⟨eij⟩, between a fixed amino acid at position i (black arrow) and all other positions j. Here, i is located at (A) the binding site, (B) the center of the channel, and (C) slightly off the channel. Underneath, the average amino acid configuration of the protein is drawn in shades of red (P) and blue (H). Significant epistasis mostly occurs along the P-rich channel, where mechanical interactions are long ranged. Although epistasis is predominantly positive, negative values also occur, mostly at the boundary of the channel (C). Landscapes are plotted for specific output site at right. L, left. (D) The two-codon correlation function Qij [5] measures the coupling between mutations at positions i and j [5]. The epistasis Eij and the gene correlation Qij show similar patterns. Axes are the positions of i and j loci. Significant correlations and epistasis occur mostly in and around the channel region (positions ∼70–130, rows 7–13).
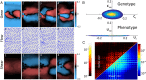
From gene to mechanical function: spectra and dimensions. (A) The first four SVD eigenvectors (in the text) of the gene Ck (Top), the displacement flow field Uk (Middle), and the shear Sk (Bottom). (B) Cross-sections through the set of solutions in the genotype space (Upper) and the phenotype space (Lower). Density of solutions is color coded. The genotype cross-section is the plane defined by the eigenvectors C3−C100, and in the phenotype space, it is defined by the eigenvectors U3−U100 (in the text). The dimensional reduction is manifested by the discoid geometry of the phenotype cloud compared with the spheroid shape of the genotype cloud. (C) Genetic correlations Qij show similarity to correlations in the shear field, s* (color coded in log scale). Corr, correlation.
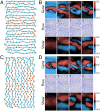
The effect of the backbone on evolution of mechanical function. The backbone induces long-range mechanical correlations, which influence protein evolution. We examine two configurations: parallel (A and B) and perpendicular (C and D) to the channel. (A and B) Parallel. (A) The backbone directs the formation of a narrow channel along the fold (compared with Fig. 5A). (B) The first four SVD eigenvectors of the gene Ck (Top), the flow Uk (Middle), and the shear Sk (Bottom). (C and D) Perpendicular. (C) The formation of the channel is “dispersed” by the backbone. (D) The first four SVD eigenvectors of Ck (Top), Uk (Middle), and shear Sk (Bottom).
Similar articles
-
Epistasis in protein evolution.
Starr TN, Thornton JW. Starr TN, et al. Protein Sci. 2016 Jul;25(7):1204-18. doi: 10.1002/pro.2897. Epub 2016 Feb 28. Protein Sci. 2016. PMID: 26833806 Free PMC article. Review.
-
How mutational epistasis impairs predictability in protein evolution and design.
Miton CM, Tokuriki N. Miton CM, et al. Protein Sci. 2016 Jul;25(7):1260-72. doi: 10.1002/pro.2876. Epub 2016 Jan 22. Protein Sci. 2016. PMID: 26757214 Free PMC article.
-
Epistasis as the primary factor in molecular evolution.
Breen MS, Kemena C, Vlasov PK, Notredame C, Kondrashov FA. Breen MS, et al. Nature. 2012 Oct 25;490(7421):535-8. doi: 10.1038/nature11510. Epub 2012 Oct 14. Nature. 2012. PMID: 23064225
-
Physical Constraints on Epistasis.
Husain K, Murugan A. Husain K, et al. Mol Biol Evol. 2020 Oct 1;37(10):2865-2874. doi: 10.1093/molbev/msaa124. Mol Biol Evol. 2020. PMID: 32421772
-
Compensatory mutations and epistasis for protein function.
Storz JF. Storz JF. Curr Opin Struct Biol. 2018 Jun;50:18-25. doi: 10.1016/j.sbi.2017.10.009. Epub 2017 Nov 5. Curr Opin Struct Biol. 2018. PMID: 29100081 Free PMC article. Review.
Cited by
-
Exploring the unfolding pathways of protein families using Elastic Network Model.
Kumar R, Dutta S. Kumar R, et al. Sci Rep. 2024 Oct 13;14(1):23905. doi: 10.1038/s41598-024-75436-8. Sci Rep. 2024. PMID: 39397155 Free PMC article.
-
Hatakeyama TS, Kaneko K. Hatakeyama TS, et al. PLoS One. 2023 Jan 26;18(1):e0277181. doi: 10.1371/journal.pone.0277181. eCollection 2023. PLoS One. 2023. PMID: 36701362 Free PMC article.
-
Simple mechanics of protein machines.
Flechsig H, Mikhailov AS. Flechsig H, et al. J R Soc Interface. 2019 Jun 28;16(155):20190244. doi: 10.1098/rsif.2019.0244. Epub 2019 Jun 19. J R Soc Interface. 2019. PMID: 31213170 Free PMC article.
-
The Statistical Trends of Protein Evolution: A Lesson from AlphaFold Database.
Tang QY, Ren W, Wang J, Kaneko K. Tang QY, et al. Mol Biol Evol. 2022 Oct 7;39(10):msac197. doi: 10.1093/molbev/msac197. Mol Biol Evol. 2022. PMID: 36108094 Free PMC article.
-
Direct coupling analysis of epistasis in allosteric materials.
Bravi B, Ravasio R, Brito C, Wyart M. Bravi B, et al. PLoS Comput Biol. 2020 Mar 2;16(3):e1007630. doi: 10.1371/journal.pcbi.1007630. eCollection 2020 Mar. PLoS Comput Biol. 2020. PMID: 32119660 Free PMC article.
References
-
- Daniel RM, Dunn RV, Finney JL, Smith JC. The role of dynamics in enzyme activity. Annu Rev Biophys Biomol Struct. 2003;32:69–92. - PubMed
-
- Bustamante C, Chemla YR, Forde NR, Izhaky D. Mechanical processes in biochemistry. Annu Rev Biochem. 2004;73:705–748. - PubMed
-
- Hammes-Schiffer S, Benkovic SJ. Relating protein motion to catalysis. Annu Rev Biochem. 2006;75:519–541. - PubMed
-
- Boehr DD, McElheny D, Dyson HJ, Wright PE. The dynamic energy landscape of dihydrofolate reductase catalysis. Science. 2006;313:1638–1642. - PubMed
Publication types
MeSH terms
Substances
LinkOut - more resources
Full Text Sources
Other Literature Sources