Building a Bird: Musculoskeletal Modeling and Simulation of Wing-Assisted Incline Running During Avian Ontogeny - PubMed
- ️Mon Jan 01 2018
Building a Bird: Musculoskeletal Modeling and Simulation of Wing-Assisted Incline Running During Avian Ontogeny
Ashley M Heers et al. Front Bioeng Biotechnol. 2018.
Abstract
Flapping flight is the most power-demanding mode of locomotion, associated with a suite of anatomical specializations in extant adult birds. In contrast, many developing birds use their forelimbs to negotiate environments long before acquiring "flight adaptations," recruiting their developing wings to continuously enhance leg performance and, in some cases, fly. How does anatomical development influence these locomotor behaviors? Isolating morphological contributions to wing performance is extremely challenging using purely empirical approaches. However, musculoskeletal modeling and simulation techniques can incorporate empirical data to explicitly examine the functional consequences of changing morphology by manipulating anatomical parameters individually and estimating their effects on locomotion. To assess how ontogenetic changes in anatomy affect locomotor capacity, we combined existing empirical data on muscle morphology, skeletal kinematics, and aerodynamic force production with advanced biomechanical modeling and simulation techniques to analyze the ontogeny of pectoral limb function in a precocial ground bird (Alectoris chukar). Simulations of wing-assisted incline running (WAIR) using these newly developed musculoskeletal models collectively suggest that immature birds have excess muscle capacity and are limited more by feather morphology, possibly because feathers grow more quickly and have a different style of growth than bones and muscles. These results provide critical information about the ontogeny and evolution of avian locomotion by (i) establishing how muscular and aerodynamic forces interface with the skeletal system to generate movement in morphing juvenile birds, and (ii) providing a benchmark to inform biomechanical modeling and simulation of other locomotor behaviors, both across extant species and among extinct theropod dinosaurs.
Keywords: avian; bird; development; flight; locomotion; musculoskeletal modeling; ontogeny; wing-assisted incline running.
Figures
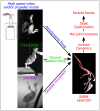
Modeling overview. Colors indicate different types of data used as simulation inputs or outputs; techniques used to collect or simulate each type of data are italicized. SIMM, Software for Interactive Musculoskeletal Modeling; XROMM, X-ray Reconstruction of Moving Morphology. The drawing of a propeller apparatus is modified from Crandell and Tobalske (2011).
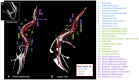
Muscles. Origins, paths, and insertions of the 30 forelimb muscles modeled. Muscles acting mainly at the shoulder are numbered in blue; muscles acting mainly at the elbow are numbered in green; muscles acting mainly at the wrist are numbered in purple. (A) adult model in anteromedial view, (B) adult model in left lateral view, (C) adult model in dorsal view.
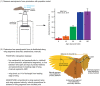
Aerodynamic force calculations. Stepwise procedure showing how the magnitudes and positions of aerodynamic forces were calculated for each model. Data in the graph is from Heers et al. (2011); the drawing of the propeller apparatus is modified from Crandell and Tobalske (2011). Resulting model inputs are shown in Table S3.
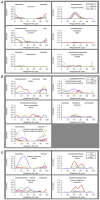
Muscle activations. Simulated patterns of muscle activation during wing-assisted incline running on 65° inclines (red, green, and purple lines) are broadly similar to the timing of muscle activity during ascending flight in pigeons (gray bars, from Dial, 1992a); for explanation of exceptions, see text. Here, the upstroke-downstroke transition is defined as the point at which the tip of the manus begins moving downward, and the downstroke-upstroke transition as the point at which the tip of the manus begins moving upward. (A) Muscles acting at the shoulder joint. (B) Muscles acting at the elbow joint. (C) Muscles acting at the wrist joint.
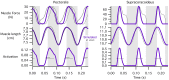
Patterns of muscle activation, changes in length, and force development. Simulation estimates of muscle activation, shortening vs. lengthening, and force development are qualitatively similar to patterns reported for flying (Tobalske and Biewener, 2008) or flap-running (Jackson et al., 2011a) pigeons. Simulated chukar data is in purple; in vivo/in vitro pigeon data is in gray; solid lines, flap-running on 65° inclines; dashed lines, ascending flight. Gray regions indicate simulated shortening of the pectoralis muscle. Lengths represent muscle-tendon lengths in chukars. Pigeon forces and lengths are expressed as stresses and strains, respectively; pigeon muscle “activity” is actually muscle excitation (EMG), which precedes activation; axes for pigeon data are not shown. All comparisons are based on adult birds.
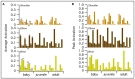
Peak and average muscle activations. On average, baby, juvenile, and adult chukars have similar levels of muscle activation (A), though the baby chukar has slightly higher peak activations than the juvenile, which has slightly higher peak activations than the adult (B). For all age classes, elbow and wrist muscles have higher average and/or peak activations than shoulder muscles, which have relatively low activations (generally <0.5) (Table S7). Each bar represents one muscle. The pectoralis and supracoracoideus are distinguished by lighter brown coloring in the top rows. P, Pectoralis; S, Supracoracoideus; C, Coracobrachialis posterior; c, Coracobrachialis anterior; Sb, Subcoracoideus; L, Latissimus dorsi; Sc, Scapulohumeralis caudalis; Ss, Subscapularis; Pb, Propatagialis brevis; D, Deltoideus major; d, Deltoideus minor; B, Biceps brachii; St, Scapulotriceps; H, Humerotriceps; Ps, Pronator sublimis; Pp, Pronator profundus; Sp, Supinator; A, Anconeus; Br, Brachialis; E, Entepicondyloulnaris; Fc, Flexor carpi ulnaris; Fs, Flexor digitorum sublimis; Fp, Flexor digitorum profundus; U, Ulnimetacarpalis ventralis; Em, Extensor metacarpi radialis; Ed, Extensor digitorum communis; Ec, Extensor carpi ulnaris; Ep, Extensor pollicis longus; Ei, Extensor indicis longus.
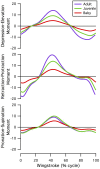
Normalized shoulder moments. Moments at the shoulder joint, standardized by body weight and moment arm lengths of the pectoralis and supracoracoideus (averaged over one wingbeat); all models were simulated under identical conditions (adult kinematics and no aerodynamic force). Joint moments increase from baby to juvenile to adult, even when accounting for body size.
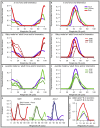
Manipulations in aerodynamic force and kinematics: effects on pectoralis (A,C,E,G,H) and supracoracoideus (B,D,F,G) activation levels. Overall, increasing aerodynamic force production to adult levels (in terms of percent body weight) in the baby and juvenile models increases activation of the pectoralis muscle during mid-downstroke, but not to high levels (<0.4, baby; <0.15, juvenile). Supracoracoideus activation decreases, because increased aerodynamic force production helps decelerate the wing and prepare for upstroke. (A,B) In vivo kinematics and aerodynamic force production; (C–F) baby or juvenile model simulated with different combinations of aerodynamic force (BF, baby force; JF, juvenile force; AF, adult force) and kinematics (BK, baby kinematics; JK, juvenile kinematics; AK, adult kinematics), in vivo activations for adult and baby or juvenile still shown; (G) in vivo kinematics with (solid lines) or without (dashed lines) aerodynamic force production, pectoralis indicated by lighter colors (red, light green, purple), and supracoracoideus by darker colors (maroon, dark green, indigo); (H) activation due to aerodynamic force production: in vivo kinematics with no aerodynamic force production subtracted from in vivo kinematics with adult aerodynamic force production, to account for ontogenetic differences in inertial properties.
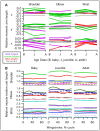
Normalized muscle moment arms and lengths. The juvenile model tends to have relatively long moment arms and muscle lengths compared to the adult and baby models. (A) Moment arms for muscles crossing the shoulder, elbow, or wrist, averaged over the stroke cycle and standardized by notarium length. Different lines are for different muscles. Red lines: moment arm is greatest (most positive or most negative) in the baby model; green: moment arm is greatest in the juvenile model; purple: moment arm is greatest in the adult model; gray: no ontogenetic trend. The juvenile has proportionally long z (elevation-depression or extension-flexion) and y (protraction-retraction or abduction-adduction) moment arms, and the adult has long x (supination-pronation) moment arms at the shoulder. (B) Muscle-tendon unit (MTU) length through one wingbeat cycle (0–100%), standardized by notarium length; different colors represent different muscles crossing the shoulder, elbow, or wrist.
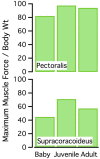
Normalized muscle force. Maximum isometric muscle force as a percentage of body weight, for the pectoralis and supracoracoideus (both sides of the body). The juvenile model has the highest maximum force, for its body size.
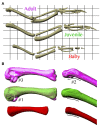
Skeletal anatomy. (A) Images of right forelimbs in dorsal view, standardized by notarium length. The proportionally long moment arms and muscle-tendon lengths (Figure 9) of the juvenile are likely due, at least partially, to its proportionally long and sometimes wide limb bones. (B) Images of right humeri (adult, purple; juvenile, green; baby, red), standardized by notarium length; left images in posterior view, right images in dorsal view. The proportionally long moment arms of the adult for long axis rotation at the shoulder (Figure 9) are at least partially due to its expanded bicipital crest (#1), exaggerated angle between the head and shaft of the humerus (#2), and expanded margo caudalis (#3).
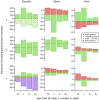
Maximum potential joint moments. Maximum potential joint moments (positive or negative muscle moment arms (in z, y, and x directions) multiplied by maximum isometric muscle force, summed for all muscles at each 1% of the stroke cycle, then averaged over the stroke cycle and normalized by notarium length and body weight) are greatest in the juvenile model for more than half of the possible joint motions. Red bars, baby has the greatest (most positive or most negative) joint moment; green bars, juvenile has the greatest joint moment; green hashed bars, juvenile and adult have similar joint moments; purple bars, adult has the greatest joint moment. Row z, elevation (+ moments) vs. depression (−) (shoulder joint) or extension (+) vs. flexion (−) (elbow, wrist); row y, protraction (+) vs. retraction (−) (shoulder) or abduction (+) vs. adduction (−) (elbow, wrist); row x, supination (+) vs. pronation (−) (all joints). B, baby model (7–8 days); J, juvenile model (18–20 days); A, adult model (>100 days) during wing-assisted incline running; AF, adult model during ascending flight, for comparison.
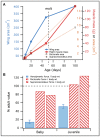
Feathers vs. muscles. (A) Wing area (blue) increases faster than muscle mass (red; all flight muscles) or physiological cross-sectional area (orange), until molt. This suggests that early in ontogeny, muscles must be “pre-equipped” to flap bigger and better wings. (B) Consistent with (A), muscles reach adult levels of performance (maximum force/body weight; red) more rapidly than wings (force/body weight, from Heers et al., ; blue), suggesting that muscles are more functionally developed than feathers in baby and juvenile chukars. Data in (A) represents two wings, and muscles on both sides of the body.
Similar articles
-
Heers AM, Baier DB, Jackson BE, Dial KP. Heers AM, et al. PLoS One. 2016 Apr 21;11(4):e0153446. doi: 10.1371/journal.pone.0153446. eCollection 2016. PLoS One. 2016. PMID: 27100994 Free PMC article.
-
Ontogeny of Flight Capacity and Pectoralis Function in a Precocial Ground Bird (Alectoris chukar).
Tobalske BW, Jackson BE, Dial KP. Tobalske BW, et al. Integr Comp Biol. 2017 Aug 1;57(2):217-230. doi: 10.1093/icb/icx050. Integr Comp Biol. 2017. PMID: 28662566
-
Aerodynamics of wing-assisted incline running in birds.
Tobalske BW, Dial KP. Tobalske BW, et al. J Exp Biol. 2007 May;210(Pt 10):1742-51. doi: 10.1242/jeb.001701. J Exp Biol. 2007. PMID: 17488937
-
New Perspectives on the Ontogeny and Evolution of Avian Locomotion.
Heers AM. Heers AM. Integr Comp Biol. 2016 Sep;56(3):428-41. doi: 10.1093/icb/icw065. Epub 2016 Jul 1. Integr Comp Biol. 2016. PMID: 27371381 Review.
-
Evolution of avian flight: muscles and constraints on performance.
Tobalske BW. Tobalske BW. Philos Trans R Soc Lond B Biol Sci. 2016 Sep 26;371(1704):20150383. doi: 10.1098/rstb.2015.0383. Philos Trans R Soc Lond B Biol Sci. 2016. PMID: 27528773 Free PMC article. Review.
Cited by
-
Kinematics and Aerodynamics of Dragonflies (Pantala flavescens, Libellulidae) in Climbing Flight.
Peng L, Pan T, Zheng M, Song S, Su G, Li Q. Peng L, et al. Front Bioeng Biotechnol. 2022 Mar 16;10:795063. doi: 10.3389/fbioe.2022.795063. eCollection 2022. Front Bioeng Biotechnol. 2022. PMID: 35372311 Free PMC article.
-
Modern three-dimensional digital methods for studying locomotor biomechanics in tetrapods.
Demuth OE, Herbst E, Polet DT, Wiseman ALA, Hutchinson JR. Demuth OE, et al. J Exp Biol. 2023 Apr 25;226(Suppl_1):jeb245132. doi: 10.1242/jeb.245132. Epub 2023 Feb 22. J Exp Biol. 2023. PMID: 36810943 Free PMC article. Review.
-
Mo A, Izzi F, Gönen EC, Haeufle D, Badri-Spröwitz A. Mo A, et al. Sci Rep. 2023 Feb 25;13(1):3290. doi: 10.1038/s41598-023-30318-3. Sci Rep. 2023. PMID: 36841875 Free PMC article.
-
Exploring the functional morphology of the Gorilla shoulder through musculoskeletal modelling.
van Beesel J, Hutchinson JR, Hublin JJ, Melillo SM. van Beesel J, et al. J Anat. 2021 Jul;239(1):207-227. doi: 10.1111/joa.13412. Epub 2021 Feb 24. J Anat. 2021. PMID: 33629406 Free PMC article.
-
Etienne C, Houssaye A, Fagan MJ, Hutchinson JR. Etienne C, et al. J Anat. 2024 Aug;245(2):240-257. doi: 10.1111/joa.14041. Epub 2024 Apr 1. J Anat. 2024. PMID: 38558391 Free PMC article.
References
-
- Anderson J. D. (2017). Fundamentals of Aerodynamics, 6th Edn. New York, NY: McGraw-Hill Education.
LinkOut - more resources
Full Text Sources