Bacterial ageing in the absence of external stressors - PubMed
- ️Tue Jan 01 2019
Bacterial ageing in the absence of external stressors
Urszula Łapińska et al. Philos Trans R Soc Lond B Biol Sci. 2019.
Abstract
Evidence of ageing in the bacterium Escherichia coli was a landmark finding in senescence research, as it suggested that even organisms with morphologically symmetrical fission may have evolved strategies to permit damage accumulation. However, recent work has suggested that ageing is only detectable in this organism in the presence of extrinsic stressors, such as the fluorescent proteins and strong light sources typically used to excite them. Here we combine microfluidics with brightfield microscopy to provide evidence of ageing in E. coli in the absence of these stressors. We report (i) that the doubling time of the lineage of cells that consistently inherits the 'maternal old pole' progressively increases with successive rounds of cell division until it reaches an apparent asymptote, and (ii) that the parental cell divides asymmetrically, with the old pole daughter showing a longer doubling time and slower glucose accumulation than the new pole daughter. Notably, these patterns arise without the progressive accumulation or asymmetric partitioning of observable misfolded-protein aggregates, phenomena previously hypothesized to cause the ageing phenotype. Our findings suggest that ageing is part of the naturally occurring ecologically-relevant phenotype of this bacterium and highlight the importance of alternative mechanisms of damage accumulation in this context. This article is part of a discussion meeting issue 'Single cell ecology'.
Keywords: ageing; bacteria; glucose uptake; protein aggregates; senescence; single-cell analysis.
Conflict of interest statement
We have no competing interests.
Figures
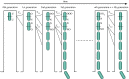
Schematics illustrating our approach for quantifying ageing in E. coli. After loading the mother machine with a stationary phase culture, we followed the bacterium at the top of each dead-end channel with unknown orientation (U) so that we could establish which one of its poles was the newer and which one the older pole. We defined T0 the time it took this bacterium to divide (not the actual doubling time of the cell as it last divided prior to being loaded in the mother machine). The two daughters of this bacterium inherit a new (N) and an old (O) pole each. We defined T1,1 and T2,1 as the doubling times of the first and second daughter from the top of the dead-end channel, respectively. Upon division the first daughter from the top gives rise to two further daughters: the daughter at the top inherits an OO and a N pole, the second daughter from the top inherits a N and a NO pole. After n generations, the first daughter from the top of the channel will have inherited (O)n and N poles and display a doubling time T1,n; the second daughter from the top of the channel will have inherited N and NO poles and display a doubling time T2,n. (Online version in colour.)
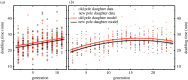
Ageing and asymmetric division in E. coli. Dependence of cell doubling time on generation number for old pole (red) and new pole daughter cells (black). (a) Old pole daughter cells took longer to divide than new pole daughter cells (LMM: χ12=5.192, p = 0.023; electronic supplementary material, table S1). For both cell types, division time progressively increased over successive generations (LMM: χ12=38.327, p < 0.001; electronic supplementary material, table S1). This analysis was carried out including data for generations 2–11 from both experimental runs (20 channels in total), controlling for variation among runs (see the electronic supplementary material, table S1 for full model description). (b) Inspecting the 24 generation dataset (run B) revealed that the progressive increase in division times gradually asymptotes to a steady state division time with increasing generation number (LMM: linear effect of generation number: χ12=52.199, p < 0.001; quadratic effect of generation number: χ12=32.832, p < 0.001; electronic supplementary material, table S2). Old pole daughter cells also took longer to divide than new pole daughter cells within this 24 generation dataset (LMM: χ12=11.222, p < 0.001; electronic supplementary material, table S2). Coloured lines and grey shaded area represent mean model predictions ± standard error (s.e.) for (a) the effect of generation number and polarity across experimental runs between generation 2 and 11 inclusive (electronic supplementary material, table S1), and (b) the effect of generation number and polarity for experimental run B between generation 2 and 24 inclusive (electronic supplementary material, table S2). Red and black dots illustrate raw data points for old pole and new pole daughter cells respectively, with darker point shading reflecting overlapping data points. (Online version in colour.)
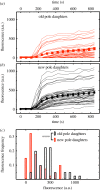
New and old pole daughters differ in their glucose uptake patterns. (a) Uptake of the fluorescent glucose analogue 2-(N-(7-Nitrobenz-2-oxa-1,3-diazol-4-yl)Amino)-2-Deoxyglucose (2-NBDG) in 40 pairs of old pole and (b) generation-matched new pole daughter cells collated from three independent mother machine experiments. Each pair was hosted in a different dead-end channel of the mother machine and constituted the 4th generation grown in the mother machine from an E. coli overnight culture. Squares and circles (and error bars) represent the means (and standard errors) of the single-cell uptake traces. 2-NBDG was introduced in the mother machine at t = 0 s. (c) At the end of each experiment (t = 840 s) new pole daughters (black empty bars) had accumulated intracellular glucose to a significantly higher level than old pole daughters (red filled bars; paired t-test, n = 40 pairs, p < 0.0001). (Online version in colour.)
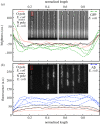
Ageing does not correlate with the formation of misfolded protein aggregates. (a) Brightness profile along the length of two pairs of old and new pole daughters at the 24th generation of growth in a mother machine seeded with an overnight E. coli culture (red dotted and black dashed lines, respectively) and of individual E. coli expressing exogenous GFP and loaded in a different mother machine from a different overnight culture (green dotted dashed lines). Only the latter exhibit significant depth in brightness in proximity of the bacterial poles resembling previously reported misfolded protein aggregates [16]. Formal statistical comparisons of brightness profiles (see §2e) showed that: (i) the wild-type E. coli cells displayed significantly less evidence of pole-associated dark foci than E. coli expressing exogenous GFP (n = 30 cells; p-value = 0.005); and (ii) wild-type E. coli old pole daughters did not show significantly stronger evidence of pole-associated dark foci than same-generation new pole daughters (a paired t-test of n = 10 pairs returned a p-value = 0.4). (b) ThT fluorescence profile along the length of two pairs of old and new pole daughters at the 24th generation of growth in a mother machine seeded with an overnight E. coli culture (red dotted and black dashed lines, respectively) and of individual E. coli expressing exogenous misfolded proteins after 4 h induction with IPTG dissolved in LB (to stimulate amyloid beta 42 production) in the mother machine from an overnight culture (blue dashed double dotted lines). Only the latter exhibit significant peaks in ThT fluorescence in proximity of the bacterial poles. Formal statistical comparisons of ThT fluorescence profiles (see §2e) revealed that: (i) the wild-type E. coli cells showed significantly less evidence of pole-associated ThT staining than E. coli expressing exogenous misfolded protein aggregates (n = 30 cells; p-value = 0.002); and (ii) wild-type E. coli old pole daughters did not show significantly stronger evidence of pole-associated ThT staining than same-generation new pole daughters (a paired t-test of n = 10 pairs returned a p-value = 0.2). (Online version in colour.)
Similar articles
-
Aging, mortality, and the fast growth trade-off of Schizosaccharomyces pombe.
Nakaoka H, Wakamoto Y. Nakaoka H, et al. PLoS Biol. 2017 Jun 20;15(6):e2001109. doi: 10.1371/journal.pbio.2001109. eCollection 2017 Jun. PLoS Biol. 2017. PMID: 28632741 Free PMC article.
-
Chao L, Rang CU, Proenca AM, Chao JU. Chao L, et al. PLoS Comput Biol. 2016 Jan 13;12(1):e1004700. doi: 10.1371/journal.pcbi.1004700. eCollection 2016 Jan. PLoS Comput Biol. 2016. PMID: 26761487 Free PMC article.
-
Protein aggregates act as a deterministic disruptor during bacterial cell size homeostasis.
Mortier J, Govers SK, Cambré A, Van Eyken R, Verheul J, den Blaauwen T, Aertsen A. Mortier J, et al. Cell Mol Life Sci. 2023 Nov 16;80(12):360. doi: 10.1007/s00018-023-05002-4. Cell Mol Life Sci. 2023. PMID: 37971522 Free PMC article.
-
Aging in bacteria, immortality or not-a critical review.
Gómez JM. Gómez JM. Curr Aging Sci. 2010 Dec;3(3):198-218. Curr Aging Sci. 2010. PMID: 20735346 Review.
-
Is Escherichia coli getting old?
Woldringh CL. Woldringh CL. Bioessays. 2005 Aug;27(8):770-4. doi: 10.1002/bies.20271. Bioessays. 2005. PMID: 16015607 Review.
Cited by
-
DeLTA 2.0: A deep learning pipeline for quantifying single-cell spatial and temporal dynamics.
O'Connor OM, Alnahhas RN, Lugagne JB, Dunlop MJ. O'Connor OM, et al. PLoS Comput Biol. 2022 Jan 18;18(1):e1009797. doi: 10.1371/journal.pcbi.1009797. eCollection 2022 Jan. PLoS Comput Biol. 2022. PMID: 35041653 Free PMC article.
-
Cama J, Voliotis M, Metz J, Smith A, Iannucci J, Keyser UF, Tsaneva-Atanasova K, Pagliara S. Cama J, et al. Lab Chip. 2020 Aug 7;20(15):2765-2775. doi: 10.1039/d0lc00242a. Epub 2020 Jul 2. Lab Chip. 2020. PMID: 32613221 Free PMC article.
-
Single-Cell Microfluidics: A Primer for Microbiologists.
Ripandelli RAA, van Oijen AM, Robinson A. Ripandelli RAA, et al. J Phys Chem B. 2024 Oct 24;128(42):10311-10328. doi: 10.1021/acs.jpcb.4c02746. Epub 2024 Oct 14. J Phys Chem B. 2024. PMID: 39400277 Free PMC article. Review.
-
Behavioural changes in slime moulds over time.
Rolland A, Pasquier E, Malvezin P, Cassandra C, Dumas M, Dussutour A. Rolland A, et al. Philos Trans R Soc Lond B Biol Sci. 2023 Apr 10;378(1874):20220063. doi: 10.1098/rstb.2022.0063. Epub 2023 Feb 20. Philos Trans R Soc Lond B Biol Sci. 2023. PMID: 36802777 Free PMC article.
-
Zhang B, Phetsang W, Stone MRL, Kc S, Butler MS, Cooper MA, Elliott AG, Łapińska U, Voliotis M, Tsaneva-Atanasova K, Pagliara S, Blaskovich MAT. Zhang B, et al. Commun Biol. 2023 Apr 14;6(1):409. doi: 10.1038/s42003-023-04745-x. Commun Biol. 2023. PMID: 37055536 Free PMC article.
References
-
- Kirkwood TBL, Holliday R. 1979. The evolution of ageing and longevity. Proc. R. Soc. Lond. B 546, 531–546. - PubMed
-
- Kirkwood TBL. 2017. The disposable soma theory. In The evolution of senescence in the tree of life (eds Shefferson R, Jones O, Salguero-Gómez R), pp. 23–39. Cambridge, UK: Cambridge University Press; (10.1017/9781139939867.002) - DOI
Publication types
MeSH terms
Grants and funding
- BB/H022716/1/BB_/Biotechnology and Biological Sciences Research Council/United Kingdom
- BB/M009122/1/BB_/Biotechnology and Biological Sciences Research Council/United Kingdom
- WT_/Wellcome Trust/United Kingdom
- MC_PC_17189/MRC_/Medical Research Council/United Kingdom
- WT097835/Z/11/Z/WT_/Wellcome Trust/United Kingdom
LinkOut - more resources
Full Text Sources
Other Literature Sources