Allosteric Modulator Leads Hiding in Plain Site: Developing Peptide and Peptidomimetics as GPCR Allosteric Modulators - PubMed
- ️Fri Jan 01 2021
Review
Allosteric Modulator Leads Hiding in Plain Site: Developing Peptide and Peptidomimetics as GPCR Allosteric Modulators
Keith M Olson et al. Front Chem. 2021.
Abstract
Allosteric modulators (AMs) of G-protein coupled receptors (GPCRs) are desirable drug targets because they can produce fewer on-target side effects, improved selectivity, and better biological specificity (e.g., biased signaling or probe dependence) than orthosteric drugs. An underappreciated source for identifying AM leads are peptides and proteins-many of which were evolutionarily selected as AMs-derived from endogenous protein-protein interactions (e.g., transducer/accessory proteins), intramolecular receptor contacts (e.g., pepducins or extracellular domains), endogenous peptides, and exogenous libraries (e.g., nanobodies or conotoxins). Peptides offer distinct advantages over small molecules, including high affinity, good tolerability, and good bioactivity, and specific disadvantages, including relatively poor metabolic stability and bioavailability. Peptidomimetics are molecules that combine the advantages of both peptides and small molecules by mimicking the peptide's chemical features responsible for bioactivity while improving its druggability. This review 1) discusses sources and strategies to identify peptide/peptidomimetic AMs, 2) overviews strategies to convert a peptide lead into more drug-like "peptidomimetic," and 3) critically analyzes the advantages, disadvantages, and future directions of peptidomimetic AMs. While small molecules will and should play a vital role in AM drug discovery, peptidomimetics can complement and even exceed the advantages of small molecules, depending on the target, site, lead, and associated factors.
Keywords: G-protein coupled receptor; allosteric modulators; biased signaling; drug discovery; nanobodies; pepducin; peptide; peptidomimetic.
Copyright © 2021 Olson, Traynor and Alt.
Conflict of interest statement
The authors declare that the research was conducted in the absence of any commercial or financial relationships that could be construed as a potential conflict of interest.
Figures
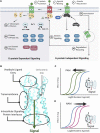
Simplified schematic of GPCR signaling pathways, AM binding sites, and positive/negative AMs. (A) Common GPCR signaling pathways are activated by an orthosteric agonist (green circle), which converts an inactive receptor (red, Ri) to an active receptor (green, Ra) (Step 1). Ra causes the transducer G-protein to exchange GDP for GTP and modulate various downstream effectors, such as kinases, ion channels, and enzymes (Step 2). Classically, signaling is terminated by phosphorylation, arrestin recruitment, and internalization (Step 3). Furthermore, arrestins can scaffold signaling pathways. Biased agonists can favor specific pathways, such as (right) preferentially stimulating arrestin signaling over (left) G-protein dependent signaling, or vice versa. (B) Classical agonists and antagonists bind to the orthosteric site (gray dotted box), as shown for Class A GPCRs. GPCR crystal structures with AMs show three general binding sites, the vestibule and ECLs in the extracellular matrix, the transmembrane region, which contacts the lipid membrane. Several different sites may exist in any of these general categories. (C) PAMs stabilize a conformation that increases orthosteric agonist affinity (α), as shown by the curve shift. Additionally, PAMs can increase the signaling efficacy (β) of orthosteric agonists (not shown). (D) NAMs decrease the potency of orthosteric agonists (as shown) by stabilizing a conformation that reduces affinity (α) or signaling efficacy (β). Figure was created with
BioRender.comand Molecular Operating Environment (MOE). PDB ID: 5C1M.A (Huang et al., 2015).
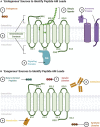
Sources to identify peptide AM leads. (A) “Endogenous” sources of peptide AMs include the following: 1) endogenous peptide sequences such as orthosteric ligands from other receptors or protein fragments; 2) nonorthosteric site residues from large endogenous bitopic peptides or hormones; 3) accessory protein protein-protein interactions, such as RAMPs, heterooligomers, and RTKs; 4) intramolecular contacts, such as sequences from the ICL (pepducins) or ECL regions; 5) signaling protein protein-protein interactions, such as Gα. (B) “Exogenous” sources for peptide AMs include 6) nature-derived peptides, such as from natural product libraries, conotoxins, cyclotides, and snake/scorpion venom; 7) genetic libraries, such as phage-display or directed evolution platforms; 8) antibodies including engineered and autoantibodies; 9) nanobodies; 10) synthetic libraries, such as combinatorial or DNA-encoded libraries. Figure was created with
BioRender.com.
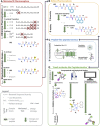
Sample workflow for peptidomimetic drug development. Sample workflow for peptidomimetic drug design of a hypothetical six-amino acid peptide lead LD1[L(1-6)]. (A) First, pharmacophore minimization occurs involving truncations of N-terminus/C-terminus and a deletion scan. In this case, the N-terminal truncation of AA1 abolished bioactivity (gray), and thus L(1-6) was retained. The C-terminal removal of AA5 and AA6 retained activity but not AA4 yielding L(1-4) or LD2. Next, deletion of the remaining amino acids is tested to further reduce the lead’s molecular weight. In the example, deleting either AA2 or AA3 abolished activity, leaving the lead unchanged L(1-4). Finally, sequential amino acid scans can identify the conformational and side-chain requirements for the pharmacophore. In the example, AA1→alanine (A) and L*-AA4 → D*-AA4 retained activity, yielded [ala1, D-AA4] L(1-4), referred to LD3. At this point, one can perform additional scans or progress to step B, C, or D. (B) The pharmacophore and bioactivity can be further refined depending on the goals. In our example, a side-chain isostere of the F3 improved bioactivity, while amide N-methylation improved metabolic stability, yielding LD4 and LD5, respectively. In principle, any modification can alter bioactivity or stability. (C) Peptide-like peptidomimetics of longer or less stable sequences can be optimized through various conjugations, such as lipidation to improve membrane permeability, macrocyclizations to stabilize the conformation and improve metabolic stability, or formulation development to make large peptides orally available. For example, lipid conjugations (black squiggly line) can improve membrane permeability of long peptides (green oval) by facilitating insertion into the membrane, followed by ‘membrane flipping’ to the intracellular surface. (D) 3-dimensionally compact pharmacophores or shorter sequences can undergo ‘small-molecule-’ like peptidomimetic development to improve desired bioactivity and druggability by stabilizing the bioactive conformation via global and local modifications, pharmacophore searches of small molecule libraries, scaffold mimetics of peptide secondary structures, or scaffold replacements using the biophysical analyses (e.g., NMR or crystallography) and computational studies (e.g., docking, conformation predictions, QSAR). Once bioactive conformation is identified, SARs iteratively (two-headed arrows) investigate the changes in activity based on structural modifications using computational or biophysical characterization. +, retained activity; ++, improved activity;−, lost activity. * indicates chirality and not a single letter amino acid. Figure was created with
BioRender.comand Molecular Operating Environment (MOE).
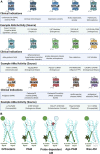
Reported AM targets for peptide/peptidomimetics therapeutics. (A) Sample of clinical indications of peptidomimetics discussed in this review. (B) AMs can produce various biological profiles. (Left) PAMs potentiate signals regardless of ligand or downstream mechanism. (Middle left) probe-dependent AMs potentiate the effects of one agonist (e.g., green) but not another agonist (e.g., light blue). (Middle right) ago-PAMs activate the receptor in the absence of an orthosteric ligand. (Right) bias-AMs preferentially activate one set of signaling pathways over another, either in the presence of an orthosteric agonist or as an ago-PAM, including but not limited to Gα versus β-arrestin signaling. Figure was created with
BioRender.com.
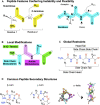
Peptide features and peptidomimetic modifications to improve their drug-like properties. (A) Peptide nomenclature dictates sequences are written left to right starting with the N-terminal labeled as "residue 1′, with each residue connected by an amide bond, i + n (left). Sites for local modifications are highlighted in yellow (right), with the peptide backbone highlighted in cyan. The peptide nomenclature and types of peptidomimetic modifications to improve drug-like properties of linear peptides include (B) local substitutions of side chains (cyan), modifications of the C- and N- termini (green), and amide bioisosteres (pink). (C) Global modifications. (D) Common secondary structures for peptides including at β-turn, a γ-turn, and (left) a α-helix top-down view or (right) α-helix side view. Dotted lines or green cylinders show backbone H-bonds. Figure was created with
BioRender.com, Chemdraw20.0, MarvinSketch, and Molecular Operating Environment (MOE).
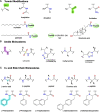
Example peptidomimetic modifications to improve activity, metabolic stability, or bioavailability of peptides. (A) Conjugation and C- and N-termini modifications generally improve peptides’ metabolic stability and bioavailability. (B) Amide bioisosteres replicate the electronic and physiochemical properties of the amide bond (pink). (C) (Top) example modifications to Cα and (bottom) sidechain isosteres of phenylalanine (F). Figure was created with Chemdraw20.0.
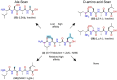
D-amino acid and alanine scans for a 5HT1B NAM—5-HT-moduline. (Left) the key pharmacophore features are highlighted in yellow as determined via an alanine scan. (Right) D-amino acid scan. (Top) examples of modifications that lost NAM function or (bottom) retained NAM activity. Structures from Plantefol et al. (1999). Uppercase letters indicate L-amino acids, and lowercase letters represent D-amino acids. The in-text compound number is bold (original name: activity). Sidechain pharmacophore features are labeled in cyan and backbone features labeled in pink. Figure was created with Chemdraw20.0.
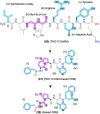
ECL-derived sequences and peptidomimetic design of NAMs and biased-PAMs at FP. The key pharmacophore features are highlighted in yellow and numbered i, ii, iii, and iv, showing the peptidomimetic development to improve bioactivity, conformational stability, and metabolic stability. The in-text compound number is bold, followed by original name: activity. Structures are originally reported by Peri et al., 2002; Bourguet et al., 2009; and Goupil et al., 2010. Sidechain pharmacophore features are labeled in cyan and backbone features labeled in pink. Figure was created with Chemdraw20.0.
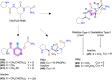
D2 AMs designed from H-PLG-NH2 to constrain a type II β-turn. Restricting the ψ2 and ϕ−3 angles stabilizing the type II β-turn improved PAM activity, and destabilization decreased PAM activity. Backbone modifications were highlighted in pink, and side-chain modifications were highlighted in cyan. The in-text compound number is bold, followed by original name: activity. Structures were originally reported by Yu et al., 1988; Sreenivasan et al., 1993; Subasinghe et al., 1993; Saitton et al., 2004; Saitton et al., 2008; and Bhagwanth et al., 2013. Figure was created with Chemdraw20.0.
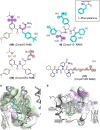
βAR2 AMs identified from exogenous synthetic libraries are polyamides with peptidomimetic-like features. (A) The β2AR cocrystallized with the PAM, Cmpd-6, bound to an intracellular binding pocket [PDB Code: 6N48 (Liu et al., 2019)]. (Inset) Side view with orthosteric ligand shown in pink. (B) The β2AR cocrystallized with the NAM, Cmpd-15, bound at an intracellular [PDB Code: 5X7D (Liu et al., 2017)]. (Inset) Side view with orthosteric ligand was shown in pink. Amide isosteres and backbone modifications are highlighted in pink; sidechain isosteres were highlighted in cyan. Van der Waals surface was shown in semitransparent surface (green = lipophilic; purple = hydrophilic). The in-text compound number is bold, followed by original name: activity. Structures were originally reported by Ahn et al., 2017 and Ahn et al., 2018. Figure was created with Chemdraw20.0 Marvin Sketch and MOE.
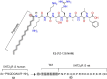
Peptide conjugations to improve physicochemical properties of pepducins at PAR1 and 5-HT2C. Example of pepducins derived for the ICL sequences that are unconjugated (acetyl) or conjugated to a palmitate- or cell-penetrating sequence (e.g., TAT). The in-text compound number is bold, followed by original name: activity. Structures were originally reported by Ji et al., 2006 and Anastasio et al., 2013. Figure was created with Chemdraw20.0, MarvinSketch, and Molecular Operating Environment (MOE).

Global conformational restraints to stabilize secondary structures with AMs identified from Gαs and conotoxins. (A) Model of stapled peptides from Gαs to stabilize αhelix based on the crystal structure (PDB Code: 3SN6) (Rasmussen et al., 2011). (B) Conotoxin AMs ρTIA at the α1-adrenoreceptor and α-conotoxin Vc1.1 at the GABAB stabilized by disulfide bonds—NMR structures ρTIA NMR (PDB Code: 2LR9) (Ragnarsson et al., 2013) and (C) NMR structure of Vc1.1 (PDB Code: 2H8S) (Clark et al., 2006). Yellow highlights residues involved in macrocyclization. * (pink letters) indicate side chains replaced with an alkyne and azide group to mediate “stapling.” Yellow indicates residues involved in macrocyclization. Key residues for bioactivity are shown in cyan. The in-text compound number is bold, followed by original name: activity. Structures were originally reported by Krajewski et al., 2001; Sharpe et al., 2003; and Boyhus et al., 2018. Figure was created with Chemdraw20.0, MarvinSketch, and Molecular Operating Environment (MOE).
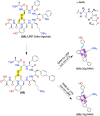
Global restraints and scaffold replacement to identify probe-dependent AMs at UTII. The disulfide bond stabilizes the γ-turn. Substitution of peptide backbone that retained key pharmacophore elements led to probe-dependent NAMs for either endogenous orthosteric agonist, URP or UTII. Cyan highlights “sidechain” differences between (61) and (62). The yellow highlights show a structural difference that leads to probe dependence. The in-text compound number is bold, followed by original name: activity. Structures were originally reported by Chatenet et al., 2013; Dufour-Gallant et al., 2015; and Douchez et al., 2017. Figure was created with Chemdraw20.0.
Similar articles
-
Shpakov AO. Shpakov AO. Int J Mol Sci. 2023 Mar 24;24(7):6187. doi: 10.3390/ijms24076187. Int J Mol Sci. 2023. PMID: 37047169 Free PMC article. Review.
-
Development of allosteric modulators of GPCRs for treatment of CNS disorders.
Nickols HH, Conn PJ. Nickols HH, et al. Neurobiol Dis. 2014 Jan;61:55-71. doi: 10.1016/j.nbd.2013.09.013. Epub 2013 Sep 27. Neurobiol Dis. 2014. PMID: 24076101 Free PMC article. Review.
-
Reinecke BA, Wang H, Zhang Y. Reinecke BA, et al. Curr Top Med Chem. 2019;19(26):2378-2392. doi: 10.2174/1568026619666191009164609. Curr Top Med Chem. 2019. PMID: 31833462 Review.
-
Hurevich M, Talhami A, Shalev DE, Gilon C. Hurevich M, et al. Curr Top Med Chem. 2014;14(15):1842-63. doi: 10.2174/1568026614666140901130843. Curr Top Med Chem. 2014. PMID: 25175995 Review.
-
Allosteric modulators of G-protein-coupled receptors.
May LT, Christopoulos A. May LT, et al. Curr Opin Pharmacol. 2003 Oct;3(5):551-6. doi: 10.1016/s1471-4892(03)00107-3. Curr Opin Pharmacol. 2003. PMID: 14559102 Review.
Cited by
-
Root-Bernstein R. Root-Bernstein R. Pharmaceuticals (Basel). 2022 Feb 10;15(2):214. doi: 10.3390/ph15020214. Pharmaceuticals (Basel). 2022. PMID: 35215326 Free PMC article. Review.
-
Olson KM, Devereaux AL, Chatterjee P, Saldaña-Shumaker SL, Shafer A, Plotkin A, Kandasamy R, MacKerell AD Jr, Traynor JR, Cunningham CW. Olson KM, et al. Front Pharmacol. 2023 Jul 3;14:1230053. doi: 10.3389/fphar.2023.1230053. eCollection 2023. Front Pharmacol. 2023. PMID: 37469877 Free PMC article.
-
Finding the Perfect Fit: Conformational Biosensors to Determine the Efficacy of GPCR Ligands.
Olson KM, Campbell A, Alt A, Traynor JR. Olson KM, et al. ACS Pharmacol Transl Sci. 2022 Aug 14;5(9):694-709. doi: 10.1021/acsptsci.1c00256. eCollection 2022 Sep 9. ACS Pharmacol Transl Sci. 2022. PMID: 36110374 Free PMC article. Review.
-
Shpakov AO. Shpakov AO. Int J Mol Sci. 2023 Mar 24;24(7):6187. doi: 10.3390/ijms24076187. Int J Mol Sci. 2023. PMID: 37047169 Free PMC article. Review.
-
Membrane Hormone Receptors and Their Signaling Pathways as Targets for Endocrine Disruptors.
Combarnous Y, Nguyen TMD. Combarnous Y, et al. J Xenobiot. 2022 Mar 25;12(2):64-73. doi: 10.3390/jox12020007. J Xenobiot. 2022. PMID: 35466213 Free PMC article. Review.
References
Publication types
LinkOut - more resources
Full Text Sources
Other Literature Sources