Persistent sodium currents in SCN1A developmental and degenerative epileptic dyskinetic encephalopathy - PubMed
- ️Fri Jan 01 2021
Persistent sodium currents in SCN1A developmental and degenerative epileptic dyskinetic encephalopathy
Kathleen M Gorman et al. Brain Commun. 2021.
Abstract
Pathogenic variants in the voltage-gated sodium channel gene (SCN1A) are amongst the most common genetic causes of childhood epilepsies. There is considerable heterogeneity in both the types of causative variants and associated phenotypes; a recent expansion of the phenotypic spectrum of SCN1A associated epilepsies now includes an early onset severe developmental and epileptic encephalopathy with regression and a hyperkinetic movement disorder. Herein, we report a female with a developmental and degenerative epileptic-dyskinetic encephalopathy, distinct and more severe than classic Dravet syndrome. Clinical diagnostics indicated a paternally inherited c.5053G>T; p. A1685S variant of uncertain significance in SCN1A. Whole-exome sequencing detected a second de novo mosaic (18%) c.2345G>A; p. T782I likely pathogenic variant in SCN1A (maternal allele). Biophysical characterization of both mutant channels in a heterologous expression system identified gain-of-function effects in both, with a milder shift in fast inactivation of the p. A1685S channels; and a more severe persistent sodium current in the p. T782I. Using computational models, we show that large persistent sodium currents induce hyper-excitability in individual cortical neurons, thus relating the severe phenotype to the empirically quantified sodium channel dysfunction. These findings further broaden the phenotypic spectrum of SCN1A associated epilepsies and highlight the importance of testing for mosaicism in epileptic encephalopathies. Detailed biophysical evaluation and computational modelling further highlight the role of gain-of-function variants in the pathophysiology of the most severe phenotypes associated with SCN1A.
Keywords: Hodgkin–Huxley model; SCN1A; epileptic encephalopathy; non-inactivating current; sodium-channel gating.
© The Author(s) (2021). Published by Oxford University Press on behalf of the Guarantors of Brain.
Figures
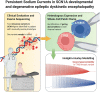
Note:Created with BioRender.com
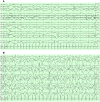
EEG aged 15 months. (A) (Awake) and (B) (sleep): EEG (HF filter, 70 Hz, sensitivity, 20 mV/mm; timebase, 30 mm/s) showing a slow awake background (mainly theta activity) and infrequent sharp waves. In sleep, epileptiform sharp waves were activated in bilateral temporal regions.
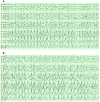
EEG aged 23 months. (A) (Awake) and (B) (sleep): EEG (HF filter, 70 Hz, sensitivity, 30 mV/mm; timebase, 30 mm/s) showing an epileptic encephalopathy with slow-wave and spike 2.5 Hz epileptiform discharges. Activation of continuous generalized epileptiform discharges in sleep.
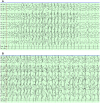
EEG aged 3 years. (A) (Atypical absence during awake state) and (B) (sleep): EEG (HF filter, 70 Hz, sensitivity, 30 mV/mm; timebase, 30 mm/s). Clinical event characterized by staring and cessation of hyperkinetic movements for 8 s with an EEG correlation of high-amplitude generalized regular 2Hz discharges. The epileptiform discharges become continuous in sleep.
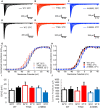
T782I and A1685S mutants in Nav1.1 produce functional channels. (A,B) Macroscopic inward currents during depolarizing steps from a holding potential of −130 mV through WT, T782I, A1685S and co-transfected T782I-A1685S Nav1.1 at 32°C and 37°C. (C) Conductance-voltage relationships for WT (black), T782I (red), A1685S (blue) and co-transfected T782I-A1685S (purple) Nav1.1 at 32°C. (D) Conductance-voltage relationships for WT, T782I, A1685S and co-transfected T782I-A1685S Nav1.1 at 37°C using the same colour scheme as (C). (E) Average voltage of half-maximal conductance for WT (black; N = 12 at 32°C; N = 13 at 37°C), T782I (red; N = 10 at 32°C; N = 13 at 37°C), A1685S (blue; N = 9 at 32°C; N = 9 at 37°C) and co-transfected T782I-A1685S (purple; N = 10 at 32°C; N = 10 at 37°C) Nav1.1 at 32°C (open symbols) and 37°C (closed symbols). Individual observations in each condition are shown as smaller symbols. (F) Average peak current at 0 mV for WT, T782I, A1685S and co-transfected T782I-A1685S Nav1.1 at 32°C and 37°C using the same colour scheme as (F). The number of observations in (F) are the same for each condition as in (F). Individual observations in each condition are shown as smaller symbols. Each midpoint measurement reflects a mean and each error bar reflects a standard error of the mean. Independent replicates are individual cells recorded in the whole-cell patch clamp configuration. * indicates a statistically significant difference between two midpoints. All statistical analysis was performed with a two-factor ANOVA followed by a Dunnett’s post hoc of pairwise comparisons to WT. Exact P-values and details of statistical tests are found in the main text. Number of individual replicates, means and standard error of the mean for all conditions are found in Table 2 and
https://doi.org/10.17605/OSF.IO/YS5RG.
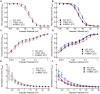
T782I and A1685S mutants in Nav1.1 minimally alter fast inactivation. (A) Fast inactivation voltage-dependence for WT (black, N = 11), T782I (red, N = 8), A1685S (blue, N = 8) and co-transfected T782I-A1685S (purple, N = 10) Nav1.1 at 32°C. (B) Fast inactivation voltage-dependence for WT (N = 12), T782I (N = 12), A1685S (N = 9) and co-transfected T782I-A1685S (N = 9) Nav1.1 at 37°C using the same colour scheme as (A). (C) Time course of fast inactivation recovery at −90 mV for WT (N = 11), T782I (N = 8), A1685S (N = 9) and co-transfected T782I-A1685S (N = 7) Nav1.1 at 32°C using the same colour scheme as (A). (D) Time course of fast inactivation recovery at −90 mV for WT (N = 11), T782I (N = 11), A1685S (N = 9) and co-transfected T782I-A1685S (N = 7) Nav1.1 at 37°C using the same colour scheme as (A). (E) Time constants of fast inactivation onset for WT (N = 11), T782I (N = 8), A1685S (N = 7) and co-transfected T782I-A1685S (N = 10) Nav1.1 at 32°C using the same colour scheme as (A). (F) Time constants of fast inactivation onset for WT (N = 13), T782I (N = 12), A1685S (N = 8) and co-transfected T782I-A1685S (N = 9) Nav1.1 at 37°C using the same colour scheme as (A). Each mid-point measurement represents a mean and each error bar represents a standard error of the mean. Independent replicates are individual cells recorded in the whole-cell patch clamp configuration. Number of individual replicates, means and standard error of the mean for all conditions are found in Table 2 and
https://doi.org/10.17605/OSF.IO/YS5RG.
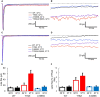
(A) Sample currents at −20 mV from WT (black), T782I (red), A1685S (blue) and co-transfected T782I-A1685S (purple) Nav1.1 at 32°C. (B) Sample non-inactivating currents at −20 mV in WT, T782I, A1685S and co-transfected T782I-A1685S Nav1.1 at 32°C using the same colour scheme as (A). (C) Sample currents at −20 mV from WT, T782I, A1685S and co-transfected T782I-A1685S Nav1.1 at 37°C using the same colour scheme as (A). (D) Sample non-inactivating currents at −20 mV in WT, T782I, A1685S and co-transfected T782I-A1685S Nav1.1 at 37°C using the same colour scheme as (A). Note the A1685S cell shown had a larger peak current than the WT cell. E) Average amplitude of non-inactivating current at −20 mV for WT (N = 8 at 32°C; N = 7 at 37°C), T782I (N = 6 at 32°C; N = 7 at 37°C), A1685S (N = 4 at 32°C; N = 5 at 37°C) and co-transfected T782I-A1685S (N = 6 at 32°C; N = 6 at 37°C) Nav1.1 at 32°C (open symbols) and 37°C (closed symbols) using the same colour scheme as (A). Individual observations in each condition are shown as smaller symbols. (F) Average fraction of non-inactivating current at −20 mV normalized to peak current for WT, T782I, A1685S and co-transfected T782I-A1685S Nav1.1 at 32°C and 37°C using the same colour scheme as (E). The number of observations in (F) are the same for each condition as in (E). Individual observations in each condition are shown as smaller symbols. Each midpoint measurement reflects a mean and each error bar reflects a standard error of the mean. Independent replicates are individual cells recorded in the whole-cell patch clamp configuration. * indicates a statistically significant difference between two midpoints. All statistical analysis was performed with a two-factor ANOVA followed by a Dunnett’s post hoc of pairwise comparisons to WT. Exact P-values and details of statistical tests are found in the main text. Number of individual replicates, means and standard error of the mean for all conditions are found in Table 2 and
https://doi.org/10.17605/OSF.IO/YS5RG.
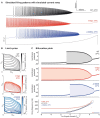
Mutant voltage-gated sodium channels confer differences in neuronal response to stimulation. (A) Single compartment neuron simulations for WT and mutant sodium channels with slowly increasing stimulating current Istim. T782I mutant channels confer hyperexcitability at low input current levels, whilst A1685S mutants allow firing even at much higher levels than WT. In the T782I-A1685S model, the effects of T782I predominate. (B) Gating variables plotting limit cycles during action potentials at different input current levels. The limit cycles appear similar for wild type and A1685S mutants, whilst T782I and T782I-A1685S do not achieve full inactivation at any input current amplitude. (C) Results from repeat simulations at different levels of input currents. Top four plots show maximum and minimum values during the simulated period at steady state, with oscillatory regimes highlighted with shading. The bottom plot shows simulated firing frequency at the different input current levels. Wildtype and A1685S mutants show threshold-like phase transitions at certain Istim values when neuronal firing is initiated. Even at the lowest input currents tested here, both T782I and T782I-A1685S simulated neurons already show fast action potential firing. At very high input currents, all simulated cells cease firing.
Similar articles
-
Brunklaus A, Brünger T, Feng T, Fons C, Lehikoinen A, Panagiotakaki E, Vintan MA, Symonds J, Andrew J, Arzimanoglou A, Delima S, Gallois J, Hanrahan D, Lesca G, MacLeod S, Marjanovic D, McTague A, Nuñez-Enamorado N, Perez-Palma E, Scott Perry M, Pysden K, Russ-Hall SJ, Scheffer IE, Sully K, Syrbe S, Vaher U, Velayutham M, Vogt J, Weiss S, Wirrell E, Zuberi SM, Lal D, Møller RS, Mantegazza M, Cestèle S. Brunklaus A, et al. Brain. 2022 Nov 21;145(11):3816-3831. doi: 10.1093/brain/awac210. Brain. 2022. PMID: 35696452 Free PMC article.
-
Miller IO, Sotero de Menezes MA. Miller IO, et al. 2007 Nov 29 [updated 2022 Feb 17]. In: Adam MP, Feldman J, Mirzaa GM, Pagon RA, Wallace SE, Amemiya A, editors. GeneReviews® [Internet]. Seattle (WA): University of Washington, Seattle; 1993–2025. 2007 Nov 29 [updated 2022 Feb 17]. In: Adam MP, Feldman J, Mirzaa GM, Pagon RA, Wallace SE, Amemiya A, editors. GeneReviews® [Internet]. Seattle (WA): University of Washington, Seattle; 1993–2025. PMID: 20301494 Free Books & Documents. Review.
-
Sodium Channelopathies in Human and Animal Models of Epilepsy and Neurodevelopmental Disorders.
Yamakawa K, Meisler MH, Isom LL. Yamakawa K, et al. In: Noebels JL, Avoli M, Rogawski MA, Vezzani A, Delgado-Escueta AV, editors. Jasper's Basic Mechanisms of the Epilepsies. 5th edition. New York: Oxford University Press; 2024. Chapter 44. In: Noebels JL, Avoli M, Rogawski MA, Vezzani A, Delgado-Escueta AV, editors. Jasper's Basic Mechanisms of the Epilepsies. 5th edition. New York: Oxford University Press; 2024. Chapter 44. PMID: 39637100 Free Books & Documents. Review.
-
Briere L, Thiel M, Sweetser DA, Koy A, Axeen E. Briere L, et al. 2023 Nov 9. In: Adam MP, Feldman J, Mirzaa GM, Pagon RA, Wallace SE, Amemiya A, editors. GeneReviews® [Internet]. Seattle (WA): University of Washington, Seattle; 1993–2025. 2023 Nov 9. In: Adam MP, Feldman J, Mirzaa GM, Pagon RA, Wallace SE, Amemiya A, editors. GeneReviews® [Internet]. Seattle (WA): University of Washington, Seattle; 1993–2025. PMID: 37956232 Free Books & Documents. Review.
-
Brashear A, Sweadner KJ, Haq I, Napoli E, Ozelius L. Brashear A, et al. 2008 Feb 7 [updated 2024 Dec 5]. In: Adam MP, Feldman J, Mirzaa GM, Pagon RA, Wallace SE, Amemiya A, editors. GeneReviews® [Internet]. Seattle (WA): University of Washington, Seattle; 1993–2025. 2008 Feb 7 [updated 2024 Dec 5]. In: Adam MP, Feldman J, Mirzaa GM, Pagon RA, Wallace SE, Amemiya A, editors. GeneReviews® [Internet]. Seattle (WA): University of Washington, Seattle; 1993–2025. PMID: 20301294 Free Books & Documents. Review.
Cited by
-
Tian M, Chen J, Li J, Pan H, Lei W, Shu X. Tian M, et al. BMC Pediatr. 2022 Apr 25;22(1):222. doi: 10.1186/s12887-022-03246-w. BMC Pediatr. 2022. PMID: 35468813 Free PMC article.
-
Widespread genomic influences on phenotype in Dravet syndrome, a 'monogenic' condition.
Martins Custodio H, Clayton LM, Bellampalli R, Pagni S, Silvennoinen K, Caswell R; Genomics England Research Consortium; Brunklaus A, Guerrini R, Koeleman BPC, Lemke JR, Møller RS, Scheffer IE, Weckhuysen S, Zara F, Zuberi S, Kuchenbaecker K, Balestrini S, Mills JD, Sisodiya SM. Martins Custodio H, et al. Brain. 2023 Sep 1;146(9):3885-3897. doi: 10.1093/brain/awad111. Brain. 2023. PMID: 37006128 Free PMC article.
-
Brain-wide circuitry underlying altered auditory habituation in zebrafish models of autism.
Wilde M, Ghanbari A, Mancienne T, Moran A, Poulsen RE, Constantin L, Lee C, Scholz LA, Arnold J, Qin W, Karle TJ, Petrou S, Favre-Bulle I, Hoffman EJ, Scott EK. Wilde M, et al. bioRxiv [Preprint]. 2024 Sep 5:2024.09.04.611137. doi: 10.1101/2024.09.04.611137. bioRxiv. 2024. PMID: 39282371 Free PMC article. Preprint.
-
Wu SN, Wu CL, Cho HY, Chiang CW. Wu SN, et al. Int J Mol Sci. 2022 Aug 21;23(16):9453. doi: 10.3390/ijms23169453. Int J Mol Sci. 2022. PMID: 36012718 Free PMC article. Review.
-
Nwosu G, Reddy SB, Riordan HRM, Kang JQ. Nwosu G, et al. Int J Mol Sci. 2022 Aug 26;23(17):9683. doi: 10.3390/ijms23179683. Int J Mol Sci. 2022. PMID: 36077081 Free PMC article. Review.
References
-
- Covanis A. Epileptic encephalopathies (including severe epilepsy syndromes). Epilepsia. 2012;53 (Suppl 4):114–126. - PubMed
-
- McTague A, Howell KB, Cross JH, Kurian MA, Scheffer IE.. The genetic landscape of the epileptic encephalopathies of infancy and childhood. Lancet Neurol. 2016;15(3):304–316. - PubMed
-
- Absoud M, Parr JR, Halliday D, Pretorius P, Zaiwalla Z, Jayawant S.. A novel ARX phenotype: Rapid neurodegeneration with Ohtahara syndrome and a dyskinetic movement disorder. Dev Med Child Neurol. 2010;52(3):305–307. - PubMed
LinkOut - more resources
Full Text Sources