One Omics Approach Does Not Rule Them All: The Metabolome and the Epigenome Join Forces in Haematological Malignancies - PubMed
- ️Fri Jan 01 2021
Review
One Omics Approach Does Not Rule Them All: The Metabolome and the Epigenome Join Forces in Haematological Malignancies
Antonia Kalushkova et al. Epigenomes. 2021.
Abstract
Aberrant DNA methylation, dysregulation of chromatin-modifying enzymes, and microRNAs (miRNAs) play a crucial role in haematological malignancies. These epimutations, with an impact on chromatin accessibility and transcriptional output, are often associated with genomic instability and the emergence of drug resistance, disease progression, and poor survival. In order to exert their functions, epigenetic enzymes utilize cellular metabolites as co-factors and are highly dependent on their availability. By affecting the expression of metabolic enzymes, epigenetic modifiers may aid the generation of metabolite signatures that could be utilized as targets and biomarkers in cancer. This interdependency remains often neglected and poorly represented in studies, despite well-established methods to study the cellular metabolome. This review critically summarizes the current knowledge in the field to provide an integral picture of the interplay between epigenomic alterations and the cellular metabolome in haematological malignancies. Our recent findings defining a distinct metabolic signature upon response to enhancer of zeste homolog 2 (EZH2) inhibition in multiple myeloma (MM) highlight how a shift of preferred metabolic pathways may potentiate novel treatments. The suggested link between the epigenome and the metabolome in haematopoietic tumours holds promise for the use of metabolic signatures as possible biomarkers of response to treatment.
Keywords: epigenetic; gene regulation; haematological malignancies; metabolite.
Conflict of interest statement
The authors have no conflict of interest to declare.
Figures
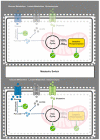
Schematic representation of the metabolic shift from oxidative phosphorylation to glycolysis. In the presence of oxygen, normal cells metabolise their glucose to pyruvate, which is then completely oxidised to CO2 and H2O via the TCA cycle and oxidative phosphorylation. Only under hypoxic conditions will normal cells reduce pyruvate to lactate through anaerobic glycolysis. Cancer cells, however, convert pyruvate into lactate, even in the presence of oxygen. In addition, while increased glucose uptake contributes to increased energy production, additional carbon sources, such as lactate and glutamine metabolism, are required to promote the synthesis of biomolecules required for rapid proliferative capacity.
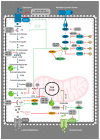
The complex regulatory network of glycolysis, lactate metabolism, and glutaminolysis is highly dependent on activation of the transcription factor HIF-1α to promote the metabolic shift from oxidative phosphorylation to glycolysis. HIF-1α participates in the glycolytic switch by regulating the expression of glucose transporters and enzymes in the glycolysis pathways. In haematological cancers, the H3K9 demethylase KDM3A contributes to an active HIF-1α phenotype and enhancement of glycolysis, while DNMT3A mediates the silencing of HIF-1α negative regulators. Additionally, miRNA regulation has a large impact on glycolysis and the access to intermediate biomolecules. MiRNAs can affect large portions of glycolysis, lactate metabolism, and glutaminolysis, which unveils a complex regulatory structure whereby miRNAs can both function as oncogenes and tumour suppressors. Red lines indicate a silencing effect. Green arrow indicates a promoting effect. All other arrows indicate enzymatic activity. GLUT1, glucose transporter type 1; HK, hexokinase; GPI, phosphoglucose isomerase; PFK, phosphofructokinase; GAPDH, G3P dehydrogenase; PGK, phosphoglycerate kinase; PGM, phosphoglyceromutase; PK, pyruvate kinase; LDH, lactate dehydrogenase; MCT4, monocarboxylate transporter 4; PDK, pyruvate dehydrogenase kinase; PDH, pyruvate dehydrogenase E1; HIF-1α, hypoxia-inducible factor 1-alpha; JMJDC, jumonji domain; SIRT4, sirtuin 4; SLC1A5, solute carrier family 1 member 5; GLS, glutaminase; GLUD, glutamate dehydrogenase; KDM3A, lysine demethylase 3A; LSD1, lysine demethylase 1A; PHD, prolyl hydroxylase domain; VHL, von Hippel-Lindau; LKB1, serine/threonine kinase 11; PTEN, phosphatase and tensin homolog; mTOR, mechanistic target of rapamycin kinase; and AKT, AKT serine/threonine kinase.
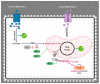
Increased glucose uptake affects the accessibility of acetyl-CoA, a key co-factor for the epigenetic regulatory machinery. Increased access to pyruvate promotes inter-mitochondrial availability, which can be used to stimulate TCA cycle activity. As mitochondrial acetyl-CoA cannot easily cross the mitochondrial membrane, active transportation of citrate from the TCA cycle is transported out of the mitochondria and converted to acetyl-CoA by ACL. High levels of acetyl-CoA promote HATs activity and can be regulated by HIF-1α by promoting activation of PDK1, which inhibits PDHs, limiting acetyl-CoA accumulation in the mitochondria. Red lines indicate inhibitory effect, while all other arrows indicate enzymatic activity. HIF-1α, hypoxia-inducible factor 1-alpha; AceCS, acetyl-CoA synthetase; PIP2, phosphatidylinositol-4,5-bisphosphate; HATs, histone acetyltransferases; PDH, pyruvate dehydrogenase E1; PDK, pyruvate dehydrogenase kinase 1; and ACL, ATP-citrate lyase.
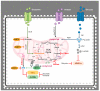
The TCA cycle is highly regulated by the access to glucose, glutamate, and acetate. Increased availability of glucose produces acetyl-CoA that can be utilised within the TCA cycle to produce citrate to be transported out of the mitochondria for acetylation conversion. In addition, increased access to glutamate allows for the TCA cycle metabolite α-ketoglutarate to accumulate, thus driving ATP production. Impairment within the TCA cycle genes has a severe impact on the epigenetic regulation. IDH2 mutation promotes accumulation of 2HG, which inhibits the TETs and JHDMs, in addition to increased levels of fumarate and succinate due to mutations in SDH or FM. Increased lactate production from pyruvate results in reduction in NAD+/NADH ratios, which inhibits SIRTs and HDACs function. Red lines indicate inhibitory effect and green arrows indicate a promoting effect, while all other arrows indicate enzymatic activity. GLS, glutaminase; GLUD, glutamate dehydrogenase; SIRT3, sirtuin 3; SIRT4, sirtuin 4; ASH1L, ASH1-like histone lysine methyltransferase; ACO, aconitase; IDH, isocitrate dehydrogenase; IDH2mt, isocitrate dehydrogenase 2 mutant; KGD, α-ketoglutarate dehydrogenase; SCS, succinyl-CoA synthase; SDHmt, succinate dehydrogenase mutant; SDH, succinate dehydrogenase; FH, fumarate hydratase; FMmt, fumarate hydratase mutant; CS, citrate synthase; PK, pyruvate kinase; PDH, pyruvate dehydrogenase E1; LDH, lactate dehydrogenase; HDAC, histone deacetylase; AceCS, acetyl-CoA synthetase; ACL, ATP-citrate lyase; 2HG, (R)-2-hydroxyglutarate; TET, Tet methylcytosine dioxygenase; and JHDM, lysine demethylase.
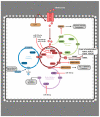
Representative illustration of the interconnection between methionine metabolism, the metabolome, and the epigenetic regulatory network. The access to methyl groups regulates the activity of histone and DNA methyltransferases. Accumulation of SAM promotes DNMTs/HMTs, while accumulation of SAH represses DNMTs/HMTs activity. A proposed miRNA-mediated silencing mechanism within methionine metabolism causes homocysteine accumulation, prevents normal cell cycle transition, and induces apoptosis in haematological malignancies. Red lines indicate inhibitory effect. 5,10-MTHF, 5, 10-methylenetetrahydrofolate; MTHFR, methylenetetrahydrofolate reductase; 5-MTHF, 5-methyltetrahydrofolic acid; MTR, 5-methyltetrahydrofolate-homocysteinemethyltransferase; THF, tetrahydrofolic acid; PHGDH, phosphoglycerate dehydrogenase; SHMT2, serinehydromethyltransferase 2; MAT2A, methionine adenosyltransferase 2A; MAT2B, methionine adenosyltransferase 2B; SAM, S-adenosylmethionine; SAH, S-adenosylhomocysteine; AHYCY, adenosylhomocysteinase; HCY, homocysteine; LAT1, L-type amino-acid transporter 1; CBS, cystathionine beta-synthase; CTH, cystathionine gamma-lyse; CYH, cystathionine; CYT, cysteine; cdk2, cycline dependent kinase 2; NNMT, nicotinamide N-methyltranferase; 1-MNA, 1-methylnicotinamide; DNMTs, DNA methyltransferases; HMTs, histone methyltransferases; AMD1, adenosylmethionine decarboxylase 1; AMA, S-adenosylmethinineamine; SRM, spermidine synthase; 5-MTA, 5′-methylthioadenosine; MTAP, methylthioadenosine phosphorylase; and PRMT5, protein arginine methyltransferase 5.
Similar articles
-
Wolahan SM, Hirt D, Glenn TC. Wolahan SM, et al. In: Kobeissy FH, editor. Brain Neurotrauma: Molecular, Neuropsychological, and Rehabilitation Aspects. Boca Raton (FL): CRC Press/Taylor & Francis; 2015. Chapter 25. In: Kobeissy FH, editor. Brain Neurotrauma: Molecular, Neuropsychological, and Rehabilitation Aspects. Boca Raton (FL): CRC Press/Taylor & Francis; 2015. Chapter 25. PMID: 26269925 Free Books & Documents. Review.
-
Metabolism and Epigenetic Interplay in Cancer: Regulation and Putative Therapeutic Targets.
Miranda-Gonçalves V, Lameirinhas A, Henrique R, Jerónimo C. Miranda-Gonçalves V, et al. Front Genet. 2018 Oct 9;9:427. doi: 10.3389/fgene.2018.00427. eCollection 2018. Front Genet. 2018. PMID: 30356832 Free PMC article. Review.
-
EZH2 as a therapeutic target for multiple myeloma and other haematological malignancies.
Tremblay-LeMay R, Rastgoo N, Pourabdollah M, Chang H. Tremblay-LeMay R, et al. Biomark Res. 2018 Dec 7;6:34. doi: 10.1186/s40364-018-0148-5. eCollection 2018. Biomark Res. 2018. PMID: 30555699 Free PMC article. Review.
-
The Epigenome in Multiple Myeloma: Impact on Tumor Cell Plasticity and Drug Response.
De Smedt E, Lui H, Maes K, De Veirman K, Menu E, Vanderkerken K, De Bruyne E. De Smedt E, et al. Front Oncol. 2018 Dec 11;8:566. doi: 10.3389/fonc.2018.00566. eCollection 2018. Front Oncol. 2018. PMID: 30619733 Free PMC article. Review.
-
Current advances of targeting epigenetic modifications in neuroendocrine prostate cancer.
Cheng WC, Wang HJ. Cheng WC, et al. Tzu Chi Med J. 2021 Feb 24;33(3):224-232. doi: 10.4103/tcmj.tcmj_220_20. eCollection 2021 Jul-Sep. Tzu Chi Med J. 2021. PMID: 34386358 Free PMC article. Review.
Cited by
-
The complex nature of lncRNA-mediated chromatin dynamics in multiple myeloma.
Nylund P, Garrido-Zabala B, Kalushkova A, Wiklund HJ. Nylund P, et al. Front Oncol. 2023 Dec 11;13:1303677. doi: 10.3389/fonc.2023.1303677. eCollection 2023. Front Oncol. 2023. PMID: 38148842 Free PMC article. Review.
-
Nylund P, Garrido-Zabala B, Párraga AA, Vasquez L, Pyl PT, Harinck GM, Ma A, Jin J, Öberg F, Kalushkova A, Wiklund HJ. Nylund P, et al. Haematologica. 2024 Feb 1;109(2):567-577. doi: 10.3324/haematol.2023.282965. Haematologica. 2024. PMID: 37496441 Free PMC article.
References
Publication types
LinkOut - more resources
Full Text Sources