Changing spatial distribution of water flow charts major change in Mars's greenhouse effect - PubMed
- ️Sat Jan 01 2022
Changing spatial distribution of water flow charts major change in Mars's greenhouse effect
Edwin S Kite et al. Sci Adv. 2022.
Abstract
Early Mars had rivers, but the cause of Mars's wet-to-dry transition remains unknown. Past climate on Mars can be probed using the spatial distribution of climate-sensitive landforms. We analyzed global databases of water-worked landforms and identified changes in the spatial distribution of rivers over time. These changes are simply explained by comparison to a simplified meltwater model driven by an ensemble of global climate model simulations, as the result of ≳10 K global cooling, from global average surface temperature [Formula: see text] ≥ 268 K to [Formula: see text] ~ 258 K, due to a weaker greenhouse effect. In other words, river-forming climates on early Mars were warm and wet first, and cold and wet later. Unexpectedly, analysis of the greenhouse effect within our ensemble of global climate model simulations suggests that this shift was primarily driven by waning non-CO2 radiative forcing, and not changes in CO2 radiative forcing.
Figures
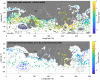
(Top) Distribution of >3.6-Ga [Late Noachian/Early Hesperian (LN/EH)] rivers (17). Color: River elevation. Gray: Excluded region (low/no detection probability). Rivers plotting within gray region are shown by black dots. Rovers: C, Curiosity at Gale crater; P, Perseverance at Jezero crater; T-1, Tianwen-1 rover (Zhurong). Elevation contour spacing is 3 km. (Bottom) Distribution of <3.6-Ga [Late Hesperian/Amazonian (LH/A)] rivers (18).
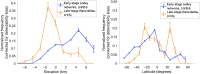
Normalized frequencies (±1σ) of the elevation (left) and latitude (right) distribution of fluvial features on early Mars (valley networks) and later Mars (fans/deltas). Figures S3 to S5 give more details and sensitivity tests.
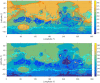
(Top) Annual average output for GCM run that best matches ~3.6-Ga data (τ = 3.14, 45° obliquity, P
co2 = 150 mbar). Color shading corresponds to annual average temperature (K). Orange line highlights 273-K isotherm, and color bands mark 10-K intervals. Dark blue and cyan contours outline cold traps. The dark blue contours contain the part of the planet’s surface area with the lowest annually integrated snow sublimation rate (0 to 10th percentile), and the cyan contours contain the part of the planet’s surface area with lower-than-average annually integrated snow sublimation rate (0 to 50th percentile). Output (64 × 48) is downscaled using MOLA topography. Elevation contours (gray) are spaced at 3-km intervals. (Bottom) As (A), but for the GCM run that best matches 3.5-Ga to 3.0-Ga data (τ = 2.46, 45° obliquity, P
co2 = 150 mbar).
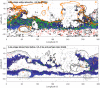
(Top) Data-model comparison for simplified snowmelt model output that best matches ~3.6-Ga data (τ = 3.1, 45° obliquity, P
co2 = 150 mbar, fsnow = 43%). Dark blue band corresponds to area of snowpack with a continuous 100-sol period with average temperature exceeding 273 K, corresponding to predicted snowmelt runoff. Green symbols correspond to true positives (mapped water-worn features within the predicted snowmelt runoff zone). Red symbols correspond to FNs (mapped water-worn features outside the predicted snowmelt runoff zone). The thick black line outlines the masked-out region (low/no detection probability for water-worn features), which differs between the two eras. Uncommon gray symbols show water-worn features within the masked-out area. Elevation contours (gray) are spaced at 3-km intervals. The orange line highlights the 273-K isotherm in annual average temperature (Youden’s J, 0.30; ROC AUC for varying fsnow for this GCM, 0.68). Rovers: C, Curiosity; P, Perseverance; T-1, Tianwen-1 rover (Zhurong). (Bottom) As (A), but for the simplified snowmelt model output that best matches 3.5-Ga to 3.0-Ga data (τ = 2.46, 45° obliquity, P
co2 = 150 mbar, fsnow = 56%) (Youden’s J, 0.34; ROC AUC for varying fsnow for this GCM, 0.65).
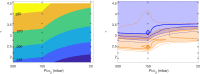
(Left) Global annual average temperature (K) as a function of P
co2 and of gray gas column optical depth, τ. Black asterisks correspond to inputs to individual GCMs, and values between the asterisks are interpolated. Obliquity = 45°. (Right) Goodness of fit of model to data as a function of P
co2 and of gray gas column optical depth, τ. Blue shaded region corresponds to relatively good fit to >3.6-Ga data, and orange shaded region corresponds to relatively good fit to <3.6-Ga data. The blue diamond is the best-fitting GCM run for >3.6-Ga data, and the orange diamond is the best-fitting GCM run for <3.6-Ga data. Metric is ROC AUC; contours correspond to 0.5, 0.55, 0.6, and 0.65 (thicker lines correspond to better fit). Asterisks correspond to inputs to individual GCMs, and values between the dots are interpolated. Figures S14 and S15 give more details and sensitivity tests.
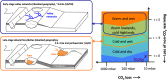
(Left column) Simple (geographically idealized) schematic of changes over time, interpreted by analysis of elevation decline (Figs. 1 and 2) in comparison to the ensemble of GCMs (Figs. 3 and 4). (Right column) Cartoon summary of Fig. 5 illustrating that, within the framework of our model, shifts can occur with or without changes in P
co2, but very probably require a decline in non-CO2 radiative forcing.
Similar articles
-
Surface Warming During the 2018/Mars Year 34 Global Dust Storm.
Streeter PM, Lewis SR, Patel MR, Holmes JA, Kass DM. Streeter PM, et al. Geophys Res Lett. 2020 May 16;47(9):e2019GL083936. doi: 10.1029/2019GL083936. Epub 2020 May 6. Geophys Res Lett. 2020. PMID: 32713983 Free PMC article.
-
Warm early Mars surface enabled by high-altitude water ice clouds.
Kite ES, Steele LJ, Mischna MA, Richardson MI. Kite ES, et al. Proc Natl Acad Sci U S A. 2021 May 4;118(18):e2101959118. doi: 10.1073/pnas.2101959118. Proc Natl Acad Sci U S A. 2021. PMID: 33903256 Free PMC article.
-
The case for a wet, warm climate on early Mars.
Pollack JB, Kasting JF, Richardson SM, Poliakoff K. Pollack JB, et al. Icarus. 1987;71:203-24. doi: 10.1016/0019-1035(87)90147-3. Icarus. 1987. PMID: 11539035
-
Subsurface water and clay mineral formation during the early history of Mars.
Ehlmann BL, Mustard JF, Murchie SL, Bibring JP, Meunier A, Fraeman AA, Langevin Y. Ehlmann BL, et al. Nature. 2011 Nov 2;479(7371):53-60. doi: 10.1038/nature10582. Nature. 2011. PMID: 22051674 Review.
-
Shakoor A, Ashraf F, Shakoor S, Mustafa A, Rehman A, Altaf MM. Shakoor A, et al. Environ Sci Pollut Res Int. 2020 Nov;27(31):38513-38536. doi: 10.1007/s11356-020-10151-1. Epub 2020 Aug 8. Environ Sci Pollut Res Int. 2020. PMID: 32770337 Review.
Cited by
-
Atmospheric oxidation drove climate change on Noachian Mars.
Liu J, Michalski JR, Wang Z, Gao WS. Liu J, et al. Nat Commun. 2024 Jul 5;15(1):5648. doi: 10.1038/s41467-024-47326-0. Nat Commun. 2024. PMID: 38969635 Free PMC article.
-
Could Life Have Started on Mars? Planetary Conditions That Assemble and Destroy Protocells.
Cary FCA, Deamer DW, Damer BF, Fagents SA, Ruttenberg KC, Donachie SP. Cary FCA, et al. Life (Basel). 2024 Mar 20;14(3):415. doi: 10.3390/life14030415. Life (Basel). 2024. PMID: 38541739 Free PMC article.
References
-
- Grotzinger J. P., Sumner D. Y., Kah L. C., Stack K., Gupta S., Edgar L., Rubin D., Lewis K., Schieber J., Mangold N., Milliken R., Conrad P. G., DesMarais D., Farmer J., Siebach K., Calef F. III, Hurowitz J., McLennan S. M., Ming D., Vaniman D., Crisp J., Vasavada A., Edgett K. S., Malin M., Blake D., Gellert R., Mahaffy P., Wiens R. C., Maurice S., Grant J. A., Wilson S., Anderson R. C., Beegle L., Arvidson R., Hallet B., Sletten R. S., Rice M., Bell J. III, Griffes J., Ehlmann B., Anderson R. B., Bristow T. F., Dietrich W. E., Dromart G., Eigenbrode J., Fraeman A., Hardgrove C., Herkenhoff K., Jandura L., Kocurek G., Lee S., Leshin L. A., Leveille R., Limonadi D., Maki J., McCloskey S., Meyer M., Minitti M., Newsom H., Oehler D., Okon A., Palucis M., Parker T., Rowland S., Schmidt M., Squyres S., Steele A., Stolper E., Summons R., Treiman A., Williams R., Yingst A.; MSL Science Team , A habitable fluvio-lacustrine environment at Yellowknife Bay, Gale crater, Mars. Science 343, 1242777 (2014). - PubMed
-
- Irwin R. P., Lewis K. W., Howard A. D., Grant J. A., Paleohydrology of Eberswalde crater, Mars. Geomorphology 240, 83–101 (2015).
-
- Grant J. A., Wilson S. A., Late alluvial fan formation in southern Margaritifer Terra, Mars. Geophys. Res. Lett. 38, L08201 (2011).
-
- Kite E. S., Geologic constraints on Early Mars climate. Space Sci. Rev. 215, 10 (2019).
-
- Ingersoll A. P., Mars: Occurrence of liquid water. Science 168, 972–973 (1970). - PubMed
LinkOut - more resources
Full Text Sources
Miscellaneous