Phenotypic plasticity for improved light harvesting, in tandem with methylome repatterning in reef-building corals - PubMed
Phenotypic plasticity for improved light harvesting, in tandem with methylome repatterning in reef-building corals
Kelly Gomez-Campo et al. Mol Ecol. 2024 Feb.
Abstract
Acclimatization through phenotypic plasticity represents a more rapid response to environmental change than adaptation and is vital to optimize organisms' performance in different conditions. Generally, animals are less phenotypically plastic than plants, but reef-building corals exhibit plant-like properties. They are light dependent with a sessile and modular construction that facilitates rapid morphological changes within their lifetime. We induced phenotypic changes by altering light exposure in a reciprocal transplant experiment and found that coral plasticity is a colony trait emerging from comprehensive morphological and physiological changes within the colony. Plasticity in skeletal features optimized coral light harvesting and utilization and paralleled significant methylome and transcriptome modifications. Network-associated responses resulted in the identification of hub genes and clusters associated to the change in phenotype: inter-partner recognition and phagocytosis, soft tissue growth and biomineralization. Furthermore, we identified hub genes putatively involved in animal photoreception-phototransduction. These findings fundamentally advance our understanding of how reef-building corals repattern the methylome and adjust a phenotype, revealing an important role of light sensing by the coral animal to optimize photosynthetic performance of the symbionts.
Keywords: DNA methylation; biological networks; biomineralization; gene expression; phenotypic plasticity.
© 2023 The Authors. Molecular Ecology published by John Wiley & Sons Ltd.
Conflict of interest statement
Competing Interest Statement: Authors declare no competing interests.
Figures
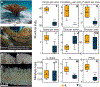
(A) A. palmata colonies have a strong intracolonial light gradient. The branching morphology exhibits modules (polyps) exposed to direct sunlight (HL surfaces) and modules growing in the shade (LL surfaces). (B) Morphological skeletal features of the branch cross-section showing the transition from upperside to underside of the branch. (C) HL surfaces and (D) LL surfaces show distinct skeletal morphology, (E) with corallites significantly taller in the surface exposed to HL. (F-N) Phenotypic traits of HL (n= 21) and LL surfaces (n=21) from 3 genets. Center lines show the median and center squares the mean; box limits indicate the 25th and 75th percentiles; whiskers extend 1 time the interquartile range. For all panels, ***P <0.001; **P <0.01; *P<0.05; nsP >0.05, two-tailed, unpaired Student’s t test. (F) Polyp density (# polyps cm−2), (G) Density of corallites larger than 3 mm in height (# polyps cm−2), (H) soluble host protein (mg protein cm−2), (I) symbiont density (# sym cm−2), (J) Chla per symbiont cell (Ci, pg Chla sym−1), (K) Chla density (mg Chla m−2), (L) photosynthetic efficiency (μmol O2 μmol quanta), (M) respiration rate (μmol O2 m−2 s-1), (N) maximum photosynthetic rate (μmol O2 m−2 s−1).
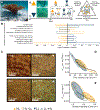
(A) Schematic representation of the experimental design. Control fragments from HL (n=7 per genet) and LL (n=7 per genet) remained unchanged, while treatment fragments (HL⇾LL, n=7 per genet; LL⇾HL, n=7 per genet) were manipulated in a reciprocal transplant that altered their light exposure by ~80%. After 13 weeks, light phenotypes were described, and tissue was collected for genomic and epigenomic analyses. (B) Fold change of main phenotypic traits showing the acclimatory mechanism to the destination light condition after 5+ weeks of transplant. (C) Visual inspection of one genet after 5+ weeks showing the change in corallite height and density. (D-E) Changes in optical traits based on specific absorption coefficients, a*Chla which describes the holobiont’s efficiency to absorb light and a*sym, which describes in hospite light absorption efficiency of the algal symbionts.
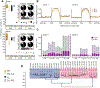
(A) Proportion of methylated cytosines (n = 8 per group condition) were highest in the CpG context and neglectable in CHG, and CHH contexts. Pie charts show the number of Cytosines (x 106) in each context. (B) Mean methylation levels of all cytosines were highest at genic regions; 2kb upstream of Transcription Start Site (TSS), and 2kb downstream of Transcription End Site (TES) are shown. Methylation levels were computed, divided to 60 bins, and plotted by genet and group condition. (C) Number of DMPs per group conditions identified by Methyl-IT, with centroid of control groups used as reference. DMPs were always higher in treatments than control samples. Two A. palmata genets are shown for comparison. (D) Hierarchical clustering of DMPs in genic regions classified by Hellinger Divergence. Classification of samples separated control (purple) and treatment (red) samples regardless of genet or destination light treatment.
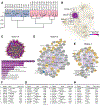
The data was prepared by combining DMG and DEG datasets to one large dataset. To estimate the initial number of possible groups we performed a (A) hierarchical clustering, which showed a classification of samples separating controls (purple) and treatments (red) groups regardless of genet or destination light treatment. (B) Whole network of gene-gene interactions. (C) Type I subnetwork showing genes with strongest gene-gene interactions (edges with strongest weights from correlation but low gene-score), denotating genes that have similar contribution to the change in phenotype (n = 199 genes). (D, E) Type II subnetworks of hub genes showing strong interactions and loadings (highest gene-scores), denotes hub genes with strongest contribution to the change in phenotype (discriminatory power of treatments from controls). (F-H) Top 10–20 genes based on gene-score in each subnetwork. The colored line between genes represents weight values from correlation matrix, low weight values (yellow) to high weight values (purple), node color indicates if DMG (yellow), both DMG-DEG (blue), DEG (grey).
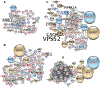
Main subnetworks of hub genes retrieved from integration of DMGs and DEGs. (A) photoreception-phototransduction (network from StringApp without attributes:
https://version11.string-db.org/cgi/network.pl?networkId=sqcU0gyKux2Y). (B) ECM-proteins, cell-cell adhesion and EGF-domains associated with soft tissue growth and calcification (network from StringApp without attributes:
https://version11.string-db.org/cgi/network.pl?networkId=8RQKxPbg9zzZ). (C) Vesicle/vacuole mediated transport, Ca2+ metabolism and cytoskeletal protein binding associated with symbiont trafficking (network from StringApp without attributes:
https://version11.string-db.org/cgi/network.pl?networkId=Cefq2PjoZN5R). (D) Innate immune response associated to interpartner recognition (network from StringApp without attributes:
https://version11.string-db.org/cgi/network.pl?networkId=pZerNp9HZxM0). Larger nodes indicate key regulators or a critical target of a regulatory pathway. The line between genes represents interactions. Node color indicates if DMG (yellow), both DMG-DEG (blue), DEG (grey). Font size represents methylation (signal density variation from Methyl-IT) and font color up (red) - down (blue) regulation. Genet 1 LL to HL are shown for interpretation.
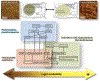
A significant change in the light environment activates photoreception mechanisms to detect cues and transduce information within cells (symbionts, cytoskeleton, extra cellular matrix-ECM, and nucleus-Nu are labeled). This activates signaling pathways to control growth, both soft tissue and skeletal growth; and in parallel, to initiate cellular transport related to symbiont recognition and changes in symbiont population densities (network from StringApp without attributes:
https://version11.string-db.org/cgi/network.pl?networkId=uh6Y1lbNXqJR).
Similar articles
-
Kruse E, Brown KT, Barott KL. Kruse E, et al. PeerJ. 2025 Jan 3;13:e18654. doi: 10.7717/peerj.18654. eCollection 2025. PeerJ. 2025. PMID: 39763704 Free PMC article.
-
Depressing time: Waiting, melancholia, and the psychoanalytic practice of care.
Salisbury L, Baraitser L. Salisbury L, et al. In: Kirtsoglou E, Simpson B, editors. The Time of Anthropology: Studies of Contemporary Chronopolitics. Abingdon: Routledge; 2020. Chapter 5. In: Kirtsoglou E, Simpson B, editors. The Time of Anthropology: Studies of Contemporary Chronopolitics. Abingdon: Routledge; 2020. Chapter 5. PMID: 36137063 Free Books & Documents. Review.
-
Hussain A, Hari Krishna Kumar S, Kumar AA, Prathiviraj R, Renjith K, Seghal Kiran G, Selvin J. Hussain A, et al. Sci Total Environ. 2025 Feb 1;963:178255. doi: 10.1016/j.scitotenv.2024.178255. Epub 2025 Jan 17. Sci Total Environ. 2025. PMID: 39824087
-
Using Experience Sampling Methodology to Capture Disclosure Opportunities for Autistic Adults.
Love AMA, Edwards C, Cai RY, Gibbs V. Love AMA, et al. Autism Adulthood. 2023 Dec 1;5(4):389-400. doi: 10.1089/aut.2022.0090. Epub 2023 Dec 12. Autism Adulthood. 2023. PMID: 38116059 Free PMC article.
-
Wilson R, Godfrey CM, Sears K, Medves J, Ross-White A, Lambert N. Wilson R, et al. JBI Database System Rev Implement Rep. 2015 Oct;13(10):146-55. doi: 10.11124/jbisrir-2015-2150. JBI Database System Rev Implement Rep. 2015. PMID: 26571290
Cited by
-
Guerrero L, Bay R. Guerrero L, et al. Evol Appl. 2024 Jul 17;17(7):e13757. doi: 10.1111/eva.13757. eCollection 2024 Jul. Evol Appl. 2024. PMID: 39027686 Free PMC article.
References
-
- Baums IB, Miller MW, & Hellberg ME (2006). Geographic variation in clonal structure in a reef-building Caribbean coral, Acropora palmata. Ecological Monographs, 76(4), 503–519. https://doi.org/1doi.org/10.1890/0012-9615(2006)076[0503:GVICSI]2.0.CO;2 - DOI
MeSH terms
LinkOut - more resources
Full Text Sources