Cell evolution and Earth history: stasis and revolution - PubMed
- ️Sun Jan 01 2006
Review
Cell evolution and Earth history: stasis and revolution
Thomas Cavalier-Smith. Philos Trans R Soc Lond B Biol Sci. 2006.
Abstract
This synthesis has three main parts. The first discusses the overall tree of life and nature of the last common ancestor (cenancestor). I emphasize key steps in cellular evolution important for ordering and timing the major evolutionary innovations in the history of the biosphere, explaining especially the origins of the eukaryote cell and of bacterial flagella and cell envelope novelties. Second, I map the tree onto the fossil record and discuss dates of key events and their biogeochemical impact. Finally, I present a broad synthesis, discussing evidence for a three-phase history of life. The first phase began perhaps ca 3.5 Gyr ago, when the origin of cells and anoxic photosynthesis generated the arguably most primitive prokaryote phylum, Chlorobacteria (= Chloroflexi), the first negibacteria with cells bounded by two acyl ester phospholipid membranes. After this 'chlorobacterial age' of benthic anaerobic evolution protected from UV radiation by mineral grains, two momentous quantum evolutionary episodes of cellular innovation and microbial radiation dramatically transformed the Earth's surface: the glycobacterial revolution initiated an oxygenic 'age of cyanobacteria' and, as the ozone layer grew, the rise of plankton; immensely later, probably as recently as ca 0.9 Gyr ago, the neomuran revolution ushered in the 'age of eukaryotes', Archaebacteria (arguably the youngest bacterial phylum), and morphological complexity. Diversification of glycobacteria ca 2.8 Gyr ago, predominantly inhabiting stratified benthic mats, I suggest caused serial depletion of 13C by ribulose 1,5-bis-phosphate caboxylase/oxygenase (Rubisco) to yield ultralight late Archaean organic carbon formerly attributed to methanogenesis plus methanotrophy. The late origin of archaebacterial methanogenesis ca 720 Myr ago perhaps triggered snowball Earth episodes by slight global warming increasing weathering and reducing CO2 levels, to yield runaway cooling; the origin of anaerobic methane oxidation ca 570 Myr ago reduced methane flux at source, stabilizing Phanerozoic climates. I argue that the major cellular innovations exhibit a pattern of quantum evolution followed by very rapid radiation and then substantial stasis, as described by Simpson. They yielded organisms that are a mosaic of extremely conservative and radically novel features, as characterized by De Beer's phrase 'mosaic evolution'. Evolution is not evenly paced and there are no real molecular clocks.
Figures
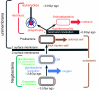
The tree of life emphasizing major revolutions in cell structure. The most basic distinction in cell biology is between negibacteria, with a cell envelope of two distinct lipid bilayer membranes, and unimembrana, with but one surface membrane. Contrary to long-standing assumptions that cells first had only one membrane, phylogenetic analysis and palaeontology jointly show that the last common ancestor of all life was a negibacterium with two surface membranes (Cavalier-Smith 1987b, 2002a, 2006a), and that unimembrana evolved from them by losing the outer membrane (OM). Within negibacteria and unimembrana two revolutions in cell-surface chemistry created radically novel bacteria whose diversification transformed the biosphere and biogeochemical cycles. The glycobacterial revolution, soon after a photosystem duplication enabling oxygenic photosynthesis, complexified the OM by adding lipopolysaccharide and novel transport machinery. Nearly 2 Gyr later, the neomuran revolution made cell surfaces potentially more flexible by replacing the rigid eubacterial corset of cross-linked murein peptidoglycan by separate glycoproteins. The neomuran revolution was closely followed by the origin of the eukaryote cell; this entailed the origin of phagotrophy, the endomembrane system and endoskeleton and enslavement of a negibacterium (specifically an α-proteobacterium) to create mitochondria (not shown: see figures 5 and 6; Cavalier-Smith 1987b, 2002c, in press). At about the same time, life belatedly colonized hot, acid environments by evolving the ancestrally hyperthermophilic archaebacteria, sisters—not ancestors—of eukaryotes; distinctly later, one archaebacterial lineage evolved biological methanogenesis. The asterisk shows a widely assumed, incorrect position for the root of the tree that was based on a few paralogue trees for a subset of proteins that underwent an episode of such extensive quantum evolution during the neomuran revolution as to fabricate that misleading position of the root (between neomura and eubacteria) by a long-branch phylogenetic reconstruction artefact (Cavalier-Smith 2002a, 2006a). Metabolic enzyme paralogue trees typically place the root within Negibacteria, but in inconsistent places (Peretó et al. 2004).
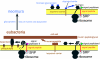
Fundamental contrasts and continuities between eubacteria and neomura in surface chemistry and protein secretion. The older eubacteria (below) typically have walls of murein peptidoglycan and lipoprotein. Neomura have a surface coat (eukaryotes) or wall (archaebacteria) of N-linked glycoprotein (oligosaccharide covalently attached to protein via asparagine: Asn). Simple surface proteins in eubacteria (lower right hexagon) and protein parts of the conjugated glycoproteins and lipoproteins have hydrophobic N-terminal signal peptides recognized by homologous signal recognition particles (SRP: grey) that then link them to SRP-receptor proteins in the membrane and transfer the signal peptide into cross-membrane protein translocation channels (not shown). Ribosomes making surface proteins temporarily stick to the membrane at ribosome receptors, forcing the growing polypeptide chain through the channel. After synthesis, signal peptidases remove signal peptides of secreted proteins: signal peptidase I, used for simple eubacterial proteins, is homologous and ancestral to the neomuran signal peptidase. The very different signal peptidase II, specific for eubacterial lipoproteins, was lost during the neomuran revolution, as were lipoproteins and murein. Hydrophilic muramopeptide precursors of eubacteria and core oligosaccharides of neomura are moved across the membrane by long-chain amphipathic isoprenol carriers (dolichol in neomura, undecaprenol in eubacteria) to which homologous enzymes temporarily attach them via N-acetylglucosamine (GlcNAc; Cavalier-Smith 2006a) before being incorporated covalently into the wall. SRPs are more complex in neomura, with a novel protein, 19P, binding to an extra helix 6 in SRP RNA that is absent from the simpler, more primitive eubacterial SRP; this extra complexity stops proteins growing further until the ribosome/SRP complex correctly docks onto the membrane, an improvement to cotranslational protein secretion associated with novel cotranslational glycoprotein synthesis in the ancestral neomuran (Cavalier-Smith 2002a). For eubacteria, the condition in Posibacteria only is shown: the more complex negibacterial envelope has an OM outside the murein (figure 3). Neomura and eubacteria also differ substantially in DNA-supercoiling machinery: in both, DNA is similarly negatively supercoiled (i.e. underwound to help strands separate in transcription and replication), but eubacteria achieve this actively by the enzyme DNA gyrase, hydrolysing ATP, whereas neomura wrap DNA passively around core histones as nucleosome particles resembling beads on a string. Replacing DNA gyrase by histones had marked coevolutionary repercussions on DNA-handling enzymes, which were substantially modified during the neomuran revolution; archaebacteria alone invented reverse gyrase by fusing two eubacterial genes, a hyperthermophilic adaptation, showing they and hyperthermophily must be more recent (Cavalier-Smith 2002a).
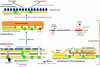
Membrane evolution and the fundamental contrast between negibacteria and posibacteria. Earliest cells were negibacteria bounded by two lipoprotein membranes: an inner cytoplasmic membrane (CM) impermeable to small hydrophilic molecules (with alpha-helical membrane proteins: green) and an OM, more permeable because of large proteinaceous pores, made by porin proteins. Between the two negibacterial membranes is the murein sacculus or cell wall: a gigantic hollow bag-shaped peptidoglycan molecule covalently cross-linked in three dimensions, rigidly enough to resist high internal osmotic pressures of more than 20 atm. The simplest negibacterial envelope (right) is in Chlorobacteria, which lack Omp85 that inserts OM β-barrel proteins in glycobacteria (left) and Hadobacteria (not shown: intermediate in complexity between chlorobacteria and glycobacteria), in both of which all known OM proteins are β-barrels. In eobacteria, the CM and OM both consist of acyl ester phospholipids plus embedded proteins. Glycobacteria retained these, but also have hopanoids that make membranes more rigid, and replaced phospholipids in the OM outer leaflet by the immensely more complex lipopolysaccharide (LPS), which so dramatically reduces OM permeability that they simultaneously evolved novel protein export machinery (using the β-barrel TolC protein) and the TonB importer of small molecules (Cavalier-Smith 2006a). For clarity, lipoproteins and Bayer's patches are not shown for glycobacteria. Posibacteria lack an OM, but have a much thicker wall of murein peptidoglycan supplemented by other polymers (teichoic acids in Endobacteria; a disparate array in Actinobacteria). Murein walls can grow and divide only by cleavage of covalent bonds by murein hydrolases. The transition from negibacteria to posibacteria involved loss of the cell wall and the origin of novel families of sortase enzymes that recognize a C-terminal LPXT motif on numerous extracellular proteins which they cleave and use for attaching them covalently to the cell wall, preventing their escape as would otherwise be likely without an OM. Posibacterial wall-attached proteins include murein lipoproteins with hydrophobic lipid tails embedded in the outer leaflet of the cytoplasmic membrane, not in the OM inner leaflet as in negibacteria.
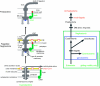
Flagellar origin unambiguously polarizes evolution from negibacteria to posibacteria. The TonB importer and its force-providing proton channel ExbB are homologues of the motor proteins MotB and MotA of eubacterial flagella and probably their evolutionary precursors (all in green); they are found in all glycobacterial phyla, including the totally non-flagellate Cyanobacteria, but never in Posibacteria or Eobacteria. The other key flagellar precursor was probably the hollow cylindrical junctional pore complex of cyanobacteria that carries a slime secretion nozzle used for gliding motility, which arguably became the flagellar basal body (Cavalier-Smith 2006a). Eubacterial flagella probably originated by novel functional association between these previously unrelated precursors: proton inflow through the MotA channel now powers basal body rotation instead of molecular import across the OM like ExcB. Both TonB and MotB are fixed to the rigid murein layer. To enable the cell to swim and not merely rotate relative to its secreted slime, it evolved the hook and shaft, made from a protein family that arose by gene duplication from unknown precursors (slime nozzle constituent?). As motor and basal body precursors are both totally absent from posibacteria, and MotB betrays a chimaeric ancestry from TonB and OM protein OmpA, also never in posibacteria, flagella cannot have arisen in a cell lacking an OM. They must have arisen in a negibacterium and been inherited by posibacteria. Therefore, posibacteria are derived from negibacteria. Evolution occurred in the direction of the arrows not its reverse. The L-ring bushing that lodges the distal end of the basal body in the OM of typical negibacteria was probably lost after the OM, when its function disappeared in the first posibacterium (Cavalier-Smith 2006a). Archaebacterial flagella are unrelated (see text); eubacterial flagella were lost with murein during the neomuran revolution. The phylogeny on the right shows the three taxa with eubacterial flagella in black; Gracilicutes include Proteobacteria and three other phyla (see figure 5). Cyanobacteria are sisters to these flagellate taxa, not their direct ancestors; therefore, the junctional pore complex evolved prior to their common ancestor.
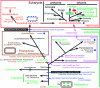
The tree of life. All 10 bacterial phyla that I currently recognize are shown, with Posibacteria split into subphyla Endobacteria and Actinobacteria (for more detailed classification see Cavalier-Smith 2002a, 2006a). It is uncertain whether Actinobacteria are paraphyletic ancestors of neomura, as shown, or their holophyletic sisters; evidence for this otherwise fully resolved bacterial phylogeny is detailed in Cavalier-Smith (2006a). Bars mark major innovations. Cortical alveoli (CA) unite Plantae and chromalveolates as corticates (Cavalier-Smith 2003b); the bikont basal trifurcation remains unresolved. Ancestrally photosynthetic taxa are in green or purple (it is likely, but not certain, that Chlorobacteria were ancestrally photosynthetic; claims for lateral transfer of photosynthesis among bacteria are unsound; see Cavalier-Smith 2006a). Chlorarachnea within Rhizaria and many euglenoids within Excavata have chloroplasts acquired by green algal enslavement; whether this happened independently (asterisks) or, as I think more likely, in a putative common ancestor of Rhizaria and Excavata (Cavalier-Smith 1999) is uncertain. For clarity, the line connecting cyanobacteria to the ancestral plastid is omitted; dashed lines show the implantation of a red algal slave (R) into the ancestral chromalveolate and mitochondria into the protoeukaryote. Secondary losses of the murein wall within Endobacteria (generating Mollicutes) and Planctobacteria are not shown: nor are more numerous losses of flagella and photosynthesis within phyla. Two hyperthermophilic eubacterial groups (Thermotogales, Aquificales) often misplaced on sequence trees, probably by long-branch attraction to the ancestrally hyperthermophilic archaebacteria, are in red. In addition to characters discussed by Cavalier-Smith (2006a), gene arrangements support the grouping Gracilicutes (including Aquifex) and exclusion of Thermotoga (Kunisawa 2006). Blue blobs mark the four taxa that include species able to make sterols. Phylogenetic arrows are not to scale—either of time or magnitude of change; only topology and the position of the root are significant. Contrary to their misleading name, Archaebacteria is the youngest bacterial phylum by many hundreds of millions of years; methanogenesis arose even later. Glycerol 1-P dehydrogenase probably evolved slightly earlier than indicated as it is also present in the eurybacterium Thermotoga.
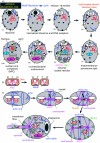
The phagotrophic origin of eukaryotes. (a) Transformation of growing trophic cells by phagotrophy and consequential endomembrane evolution. (i) A potentially flexible surface coat of N-linked glycoproteins facilitated the origin of phagocytosis by their adhesion to prey and modifying the MreB cortical skeleton (Gitai et al. 2004) into an actin skeleton able to soften locally by filament severing and form engulfing pseudopodia by localized polymerization nucleated by Arp2/3 (actin-related proteins that arose with actin by gene triplication of MreB in the pre-eukaryote; Bretschneider et al. 2004). (ii) Inevitably, internalized food vacuoles would bear attached ribosomes and sometimes also chromosomal DNA. (iii) After digestion, internalized membrane recycled by refusion with the cell surface, so initial internalization of DNA and ribosomes was reversible and impermanent. (iv) A permanent endomembrane system formed by the origin of coated vesicle budding from the internalized membrane, plus membrane return to the surface by fusion with it of transport vesicles produced by their uncoating, instead of reverse fusion of the whole food vacuole (Cavalier-Smith 2002b). Coated vesicle budding, selective for which membrane proteins are included in the budded vesicle, was a selective valve that indirectly caused fundamental differentiation between protoendomembranes and cell surface: continued phagocytosis inevitably rapidly removed all ribosome receptors from the surface, so after non-specific refusion of food vacuoles ceased it could never regain ribosomes or DNA. (v) As bacterial cell-surface derivatives, the now-permanent internal membranes had two protein-insertion mechanisms: SRP/ribosome receptors (figure 2) for cotranslationally inserting unfolded nascent proteins and the twin-arginine translocase (TAT) system for post-synthesis export of folded mature proteins. By chance these segregated into different vesicles; those with SRP receptors became protoendomembranes; those with TAT machinery became peroxisomes (P), whose membrane-embedded pex proteins import native proteins tagged by a C-terminal sequence like that recognized by TAT. (vi) Gene duplications multiplied vesicle coat types, and also the SNARE proteins whose complementary interactions ensure docking specificity onto target membranes, differentiating endomembranes into topologically, chemically and functionally distinct compartments: copI and retromers for retrograde membrane recycling from protoGolgi and endosomes; clathrin for generating endosomes from the cell surface and lysosome precursors from the trans-Golgi. (vii) An ingested α-proteobacterium (M), probably photosynthetic, escaped from its food vacuole by accidental breakage of its membrane, and multiplied in the cytoplasm. The host enslaved it by inserting inner membrane carriers that tapped its photosynthesate for host use and evolving a novel protein-targeting system; carriers probably originated from the peroxisome ATP-importer, implying that mitochondrial enslavement postdated the phagotrophy-dependent autogenous origin of peroxisomes; all theories for the host being a prokaryote are unsound. Enslavement could have started as early as shown, but the major gene transfers into host chromosomes probably postdated the nuclear envelope (Cavalier-Smith in press). (viii) Endoplasmic reticulum (ER) cisternae attached to heterochromatin via the nuclear lamina to protect DNA from shearing damage by new cytoplasmic motors (Cavalier-Smith 2005); evolution of nuclear pore complexes from the same novel eukaryotic gene family as vesicle coats (Devos et al. 2004) prevented lethal complete fusion; plugging their lumen and novel nucleocytoplasmic transport proteins excluded ribosomes, allowing mitochondrial group II introns transferred to host DNA to become spliceosomal introns only afterwards (Cavalier-Smith 1991b)—not before as Martin & Koonin (2006) mistakenly suggest. (b) Logic of the bacterial cell cycle involves DNA attachment to the cell surface by proteins and membrane division between attachment sites (Cavalier-Smith 1981, 1987b). Replication origins (O) separate by moving along a linear MreB track (Gitai et al. 2005a,b). Division of the cell membrane by a GTPase FtsZ ring is precisely between the membrane attachment points of DNA replication termini (T). (c) Origin of eukaryotic cell division, cytoskeleton and cilia. (i) Conversion of linear MreB filaments to Arp2/3-nucleated branched actin filaments and phagotrophic internalization of membranes bearing DNA negated MreB-based chromosome segregation and the FtsZ ring division mechanism, causing mis-segregation and daughters with several or (worse) no chromosomes. A new division mechanism by a ring of overlapping actin filaments nucleated by formins (Ingouff et al. 2005) evolved, but also needed positioning between the chromosomes (1) not to one side (2) to avoid wasteful DNA-less daughters. (ii) Freed from the cell surface and stabilizing selection for the now useless function of marking the surface septation site, FtsZ genes triplicated, yielding γ-tubulin for centrosomes and α- and β-tubulins for microtubules to push them apart by polymerization. 10 nm septin filaments evolved from a eubacterial GTPase to position actin rings. (iii) Kinesin evolved from an early myosin to cross-link spindle microtubules and actively slide them to separate centrosomes and attached chromosomes. (iv) Dynein evolved from an AAA ATPase to pull chromosomes (attached to microtubule minus ends by protocentromeres) and protonuclei along microtubules towards centrosomes and to separate astral microtubules. (v) Ciliary transition fibres evolved, laterally attaching a microtubule ring to the surface membrane, so their polymerization evaginated it as a protocilium; further duplications generated δ- and ϵ-tubulins, making centriolar triplets to rigidify the ciliary base. A novel kinesin (II) transported precursors into the ciliary compartment, helped by intraciliary transport particles of proteins related to vesicle coat and nuclear pore proteins; homologous α-helical-solenoid/β-sheet-propeller proteins of these three macromolecular complexes, never found in bacteria, refute theories of symbiogenetic origins of nuclei or cilia (Jekély & Arendt 2006). Numerous dynein duplications generated ciliary doublet arms, causing sliding and ciliary bending. Cytoplasmic dyneins, kinesins and myosins evolved to move vesicles and organelles along the new interphase cytoskeleton and develop cell polarity. Once centromere-based DNA segregation was efficient, mutation pressure linearized the chromosome and made multiple chromosomes and replicons per chromosome (Cavalier-Smith 1981, 1987b), while meiosis arose to correct polyploidization from residual segregation errors (Cavalier-Smith 2002b,c). The first eukaryote probably inherited cell differentiation programmes and resistant walled exospores (called cysts in protozoa) from actinobacteria; sexual cell fusion evolved prior to encystment to provide more resources to survive starvation (Cavalier-Smith 2002b).
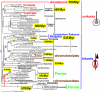
Distance tree for 58 eukaryotes based on 2994 nucleotides of 28S rRNA. This weighted least squares power 2 (GTR+Γ+I model: a=0.59231, i=0.24389) tree is from von der Heyden (2004). Numbers at major nodes only from 500 wl2 bootstrap samplings (limited to 10 min per replicate) (upper) and from 1000 BioNJ replicates (lower) are percentages of recovery of that clade (asterisks indicate 100%). Dates are for the earliest unambiguous fossils; if not otherwise obvious, dated clades are marked by yellow-filled open circles. The great variability in branch length shows that rRNA evolution is extremely non-clock-like; the still-longer-branch Amoebozoa, Foraminifera, Euglenozoa and Metamonada were omitted to reduce tree distortion and increase legibility; the position of Amoebozoa based on other evidence is shown in red. Foraminifera and Radiozoa (non-phaeodarian radiolaria) constitute the phylum Retaria, which is probably sister to Cercozoa (Cavalier-Smith 1999, 2003b). As in most single-gene trees, the monophyly of Plantae, chromalveolates and Apusozoa is not recovered. Red algae (Rhodophyta) are too low on this tree because of their very long branch, and are really sisters of Viridaeplantae (red arrow); as previously suggested for 18S rRNA (Cavalier-Smith et al. 1996), the gross acceleration in 28S rRNA evolutionary rate could have been entirely in the stem of the florideophyte red algal tree (bangiophytes have relatively normally short branches on 18S rRNA; also true for recent 28S sequences, unavailable for this analysis) as its branches have a phylogenetic depth comparable to their green-plant sisters. Thus, the ancestral florideophyte ribosome probably underwent marked episodic quantum evolution, somewhat less extreme than in the long stems at the base of the eukaryote and neomuran clades that gave rise to the misleading three-domain concept of the tree of life (Cavalier-Smith 2002a). The thumbnail sketches show that in addition to ciliary transformation from younger anterior to dissimilar older posterior organelles, bikonts have a microtubular cytosketeton (red) of cortical band ciliary roots, not a simple cone of separate microtubules like unikonts.
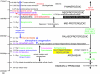
Key events in global history, integrating evidence from palaeontology and cell phylogeny. The Late Archaean and Late Proterozoic saw momentous global changes caused fundamentally by two biological revolutions—the glycobacterial and neomuran. Most phyla probably originated in bursts of adaptive radiation and quantum evolution relatively soon after these major innovations. Several new physiologies thereby created dramatically changed biogeochemical cycles. Except for a probable delay in eukaryote diversification caused by the Neoproterozoic snowball Earth episodes, their timing was probably not affected by environmental factors but by their inherent evolutionary difficulty; adaptive zones for the new phyla were created for the first time by their novel body plans, e.g. water splitting (oxygenic) photosynthesis by cyanobacteria and their immediate ancestors, phagotrophy by eukaryotes. By contrast the two major mass extinctions led to substantial lower level (class or order) innovations by removing competitors from swathes of previously occupied niches. Extinction of acritarchs probably allowed dinoflagellates, haptophytes, and later diatoms to evolve. The time of origin of Chlorobacteria (and therefore when the age of Eobacteria began) is very uncertain—any time from ca 2.9 to 3.5 Gyr, with a date within 3.1–3.5 Gyr ago being slightly preferred (see text).
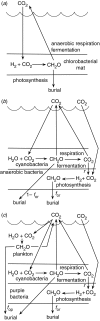
Ecosystems and the carbon cycle before and after the glycobacterial revolution. (a) In the age of chlorobacteria, life was mainly restricted to low diversity microbial mats protected from UV radiation by mineral particles. (b) After cyanobacteria evolved, mats became more complex with upper aerobic and lower anaerobic layers increasing the scope for internal CO2 recycling, with serial 13C isotope depletion by Rubisco. Isotopic composition of buried organic carbon depends on the fraction, r, of once depleted CO2 used by the second stage and on the fraction for of total C buried coming from second-stage organisms. (c) After flagella and proteobacteria evolved, they displaced chlorobacteria from most habitats and began to form a facultatively aerobic photosynthetic plankton, whose quantitative contribution greatly expanded with the ozone layer as UV inhibition declined and cyanobacterial phytoplankton evolved, especially prochlorophytes adapted to higher light intensities. As the fraction of buried organic carbon coming from plankton, fop, increased, that from recycling within the mat (for) became quantitatively insignificant from Mid-Proterozoic to Phanerozoic, thus abolishing the ultralight carbon signal of stage (b).
Similar articles
-
Cavalier-Smith T. Cavalier-Smith T. Int J Syst Evol Microbiol. 2002 Jan;52(Pt 1):7-76. doi: 10.1099/00207713-52-1-7. Int J Syst Evol Microbiol. 2002. PMID: 11837318 Review.
-
The phagotrophic origin of eukaryotes and phylogenetic classification of Protozoa.
Cavalier-Smith T. Cavalier-Smith T. Int J Syst Evol Microbiol. 2002 Mar;52(Pt 2):297-354. doi: 10.1099/00207713-52-2-297. Int J Syst Evol Microbiol. 2002. PMID: 11931142 Review.
-
Cavalier-Smith T. Cavalier-Smith T. Cold Spring Harb Perspect Biol. 2014 Sep 2;6(9):a016006. doi: 10.1101/cshperspect.a016006. Cold Spring Harb Perspect Biol. 2014. PMID: 25183828 Free PMC article. Review.
-
Hamilton TL, Bryant DA, Macalady JL. Hamilton TL, et al. Environ Microbiol. 2016 Feb;18(2):325-40. doi: 10.1111/1462-2920.13118. Epub 2015 Dec 21. Environ Microbiol. 2016. PMID: 26549614 Free PMC article. Review.
-
Origins of the machinery of recombination and sex.
Cavalier-Smith T. Cavalier-Smith T. Heredity (Edinb). 2002 Feb;88(2):125-41. doi: 10.1038/sj.hdy.6800034. Heredity (Edinb). 2002. PMID: 11932771 Review.
Cited by
-
AAA+ Ring and linker swing mechanism in the dynein motor.
Roberts AJ, Numata N, Walker ML, Kato YS, Malkova B, Kon T, Ohkura R, Arisaka F, Knight PJ, Sutoh K, Burgess SA. Roberts AJ, et al. Cell. 2009 Feb 6;136(3):485-95. doi: 10.1016/j.cell.2008.11.049. Cell. 2009. PMID: 19203583 Free PMC article.
-
Shih PM, Matzke NJ. Shih PM, et al. Proc Natl Acad Sci U S A. 2013 Jul 23;110(30):12355-60. doi: 10.1073/pnas.1305813110. Epub 2013 Jun 17. Proc Natl Acad Sci U S A. 2013. PMID: 23776247 Free PMC article.
-
Molecular paleontology and complexity in the last eukaryotic common ancestor.
Koumandou VL, Wickstead B, Ginger ML, van der Giezen M, Dacks JB, Field MC. Koumandou VL, et al. Crit Rev Biochem Mol Biol. 2013 Jul-Aug;48(4):373-96. doi: 10.3109/10409238.2013.821444. Crit Rev Biochem Mol Biol. 2013. PMID: 23895660 Free PMC article. Review.
-
Palaeoclimates: the first two billion years.
Kasting JF, Ono S. Kasting JF, et al. Philos Trans R Soc Lond B Biol Sci. 2006 Jun 29;361(1470):917-29. doi: 10.1098/rstb.2006.1839. Philos Trans R Soc Lond B Biol Sci. 2006. PMID: 16754607 Free PMC article. Review.
-
Kutschera U, Niklas KJ. Kutschera U, et al. Theory Biosci. 2008 Aug;127(3):277-89. doi: 10.1007/s12064-008-0046-8. Epub 2008 Jun 26. Theory Biosci. 2008. PMID: 18581157
References
-
- Acaso C, Wierzchos J, Speranza M, Gutiérrez J.C, González A.M, de Los Ríos A, Alonso J. Fossil protists and fungi in amber and rock substrates. Micropalaeontology. 2005;51:59–62. 10.2113/51.1.59 - DOI
-
- Alexopoulos C.J. Wiley; New York, NY: 1952. Introductory mycology.
-
- Anbar A.D, Knoll A.H. Proterozoic ocean chemistry and evolution: a bioinorganic bridge? Science. 2002;297:1137–1142. 10.1126/science.1069651 - DOI - PubMed
-
- Armstrong H.A, Brasier M.D. 2nd edn. Blackwell Scientific Publications; Oxford, UK: 2005. Microfossils; p. 296.
-
- Balch W.E, Magrum L.J, Fox G.E, Wolfe R.S, Woese C.R. An ancient divergence among the bacteria. J. Mol. Evol. 1977;9:305–311. 10.1007/BF01796092 - DOI - PubMed
Publication types
MeSH terms
LinkOut - more resources
Full Text Sources
Miscellaneous